Introduction
Acute leukemia (AL) is the leading cause of death among children in Mexico and the rest of the world1. A new epidemiological health metric reflecting global tumor burden through the years lost due to disease or disability, and considering the life expectancy of each region, has placed Latin America as one of the regions where children lose more years of life due to leukemia2. This type of neoplasm starts and progresses in the bone marrow, the tissue where hematopoiesis occurs, with the resulting imbalance in the formation of all blood cell types due to tumor growth3. Pediatric AL can occur in the lymphoid hematopoietic lineage, where B-cell precursor acute lymphoblastic leukemia (B-ALL) predominates, followed by the less frequent but very high-risk types T-cell precursor acute lymphoblastic leukemia (T-ALL) and acute myeloid leukemia (AML) of myeloid origin. Thanks to multi-institutional research programs, new conditions have been discovered recently, such as leukemia of ambiguous lineage, early T-cell precursor (ETP) leukemia, and Philadelphia chromosome-like (+) leukemia (Ph-like ALL). The knowledge of their complete identity results from the morphological analysis, karyotyping, immunophenotyping, chromosomal aberrations, transcriptomic analysis, and detection of mutations. Moreover, several clinical subgroups are now recognizable due to proteomic analysis of the surface of leukemic blasts and genomic studies that allow identifying the main differences between malignant clones and normal cell populations, revealing the high intra- and inter-tumor heterogeneity that characterizes the pathobiology of this group of diseases4,5.
In this review, we will focus on the leading cellular and molecular markers of utility as a guide for the diagnosis and tracking of minimal/measurable residual disease (MRD) by the latest generation of multiparametric flow cytometry (more than eight colors) and by molecular techniques such as quantitative reverse transcriptase-polymerase chain reaction (RT-qPCR), digital PCR (dPCR), massive next-generation sequencing (NGS), as well as their prognostic value in clinical practice.
Etiopathology of acute leukemia
The uncontrolled proliferation of oligoclonal precursors (lymphoid or myeloid) is the common feature of these diseases. According to Lapidot et al., a cell subpopulation with stemness propertiescancer stem cellsin adult myeloid leukemia gives rise to and maintains the tumor load when transplanted into immunodeficient mice6. In contrast, Ie Visuer et al. reported that this does not appear to be the case in lymphoblastic leukemia, as stemness has no obvious phenotype7, but the existence of functional leukemia-initiating cells (LICs) has been extensively documented8,9. Although the etiology of the disease is still uncertain and variable, the existence of cells that acquire stemness properties to establish and maintain the disease is undoubted. In investigating the origin, the differentiation pathways involved, and clinical diagnosis, flow cytometry has been fundamental for determining the compromised lineage, identifying the aberrant expression of specific markers and tracing them throughout the disease.
Moreover, hematopoietic differentiation maps have been constructed through this tool and functional assays in vitro and in vivo, and new markers defining the function of progenitor cells in health and disease have been discovered. Thus, the compartment that enriches stem and progenitor cell populations is CD34+CD38- with the absence of mature lineage markers (Lin-)3. The high frequency of CD34+CD38- cells at diagnosis has been associated with unfavorable outcomes and increased risk of relapse10. Under leukemic conditions, the normal progenitor compartment is numerically and functionally reduced, causing severe pancytopenia that clinically includes anemia, recurrent infections, and petechiae3,11. However, it is still a challenge to distinguish LICs from healthy hematopoietic stem cells phenotypically.
The competition between leukemic growth and normal cell development is under intense investigation. Among the most important findings is the tumor microenvironment and the remodeling of normal niches, that is, the sites where stem cells and progenitors inhabit12. It is suggested that leukemic clones produce cytokines, inflammatory factors13, and exosomal microvesicles14 containing micro RNAs and other products. These cell products drive the conditioning of normal niches and form hostile sites for normal clones favoring their escape or depletion while creating optimal niches for the proliferation of malignant clones12.
Clinical diagnosis and laboratory evidence
The oncologic treatment depends on the diagnosis, which should be comprehensive and include morphologic criteria, karyotyping, immunophenotyping, and the presence of confirmed genetic alterations. According to the World Health Organization (WHO), bone marrow aspirates should be used for leukemia diagnosis (Fig. 1). However, on certain occasions, the diagnosis can be implemented in peripheral blood as well15. Other criteria such as age, leukocyte count, evidence of infiltration to other organs, and early response to therapy help identify standard risk from high-risk patients.
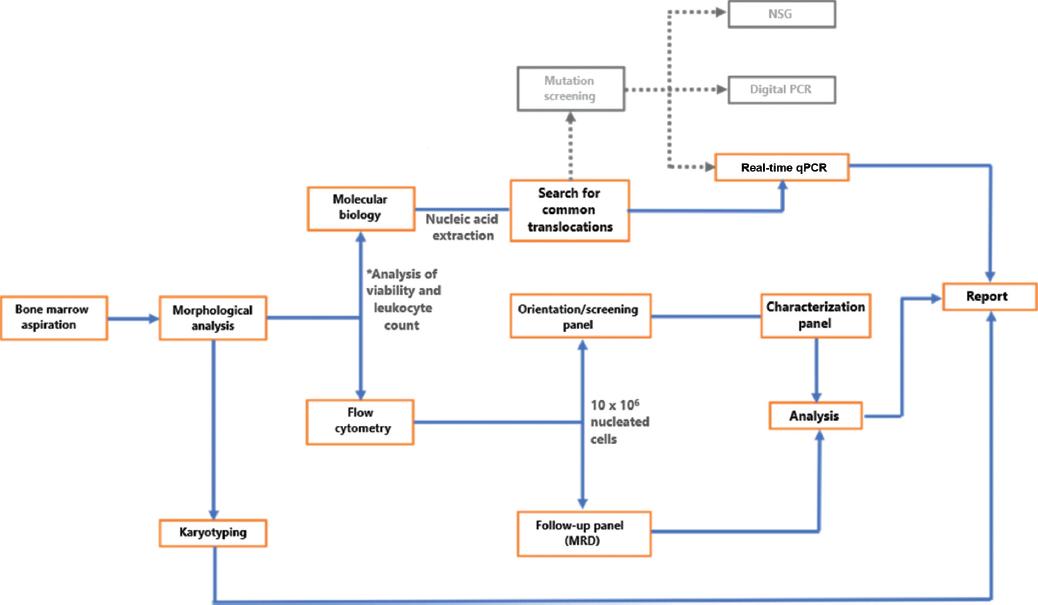
Figure 1 Workflow diagram for the comprehensive diagnosis of acute leukemia. According to the World Health Organization recommendations, leukemia diagnosis should be made from bone marrow aspirates, although peripheral blood smears can be used in particular situations. Collectively, morphological analysis, karyotyping, immunophenotyping, and molecular testing for the presence of genetic aberrations outline the comprehensive diagnosis.
The lineage suspicion is clarified by immunophenotyping, which should be initiated with a robust strategy to detect at least whether the affected lineage is lymphoid (B or T) or myeloid. If morphology yields more data on the myeloid precursor type, subsequent antibody combination or characterization panels should be based on these aspects to avoid performing lymphoid panels on clearly myeloid leukemia and vice versa16. The most commonly used antigens for the identification of B-cell lymphoid leukemia are CD19 or cytoplasmic CD79a (cyCD79a), while for T-lineage leukemia are surface CD3 (smCD3), cytoplasmic (cyCD3), and CD7, as well as the absence of antigens associated with the opposite lineage. On screening panels, myeloid leukemia is identified by the absence of the previously mentioned lymphoid antigens and the presence of myeloperoxidase (MPO) or some myeloid membrane antigens, including CD33, CD14, or CD13. In normal hematopoiesis, the common leukocyte antigen CD45 is acquired with maturation; thus, its low expression or absence is associated with more than 90% of AL cases and allows a clear identification of immature populations. The CD34 antigen, associated with stem cells and hematopoietic progenitors, is widely used in various diagnostic panels and denotes the level of (in) differentiation of leukemic blasts. In parallel, the search for translocations is usually performed routinely through commercial kits that can detect up to 28 leukemia-associated translocations: del1 (p32) (STIL-TAL1), t(1;11) (p32;q23) (MLL-EPS15), t(1;11) (q21;q23) (MLL-MLLT11), t(1;19) (q23;p13) (TCF3-PBX1), t(3;5) (q25;q34) (NPM1-MLF1), t(3;21) (q26;q22) (RUNX1-MECOM), t(4;11) (q21;q23) (MLL-AFF1), t(5;12) (q33;p13) (ETV6-PDGFRB), t(5;17) (q35;q21) (NPM1-RARA), t(6;9) (p23;q34) (DEK-NUP214), t(6;11) (q27;q23) (MLL-MLLT4), t(8;21) (q22;q22) (RUNX1-RUNX1T1), t(9;9) (q34;q34) (SET-NUP214), t(9;11) (p22;q23) (MLL-MLLT3), t(9;12) (q34;p13) (ETV6-ABL1), t(9,22) (q34;q11) (BCR-ABL1), t(10;11) (p12;q23) (MLL-MLLT10), t(11;17) (q23;q21) (MLL-MLLT6), t(11;17) (q23;q21) (ZBTB16-RARA), t(11;19) (q23;p13.1) (MLL-ELL), t(11;19) (q23;p13.3) (MLL-MLLT1), t(12;21) (p13;q22) (ETV6-RUNX1), t(12;22) (p13;q11) (ETV6-MN1), t(15;17) (q24;q21) (PML-RARA), inv(16) (p13;q22) (CBFB-MYH11), t(16;21) (p11;q22) (FUS-ERG), t(17;19) (q22;p13) (TCF3-HLF), and t(X;11) (q13;q23) (MLL-FOXO4)17.
Only 20% of B-lineage leukemia and 60% of myeloid leukemia have common translocations18. However, more than 200 aberrations associated with this group of diseases are being explored through massive sequencing, real-time quantitative reverse transcription PCR (RT-qPCR), and digital PCR strategies, although they are only available for research. Notably, no other prognostic factor has been described with such a high significance as the ultrasensitive detection of MRD for AL through high-resolution technological systems. In addition, sensitivity is a highly relevant subject that involves technological aspects, reproducible protocols, systematized analysis strategies, and high technical resolution power. We describe the main benefits of several technologies applied to the diagnosis and evaluation of residual leukemia.
MRD and its prognostic value
Once the diagnosis is established, the next step is to monitor the disease and the effectiveness through the detection of MRD. Several studies have emphasized the usefulness of monitoring tumor burden throughout treatment. Depending on the protocol, MRD can be determined on days 14, 21, 28, 33, or up to 78 days after treatment. The first attempts to monitor the reduction of malignant cells were carried out by observing histology slides and conventional staining. The introduction of terminal deoxynucleotidyl transferase (TdT) detection by fluorescence microscopy was of limited utility compared to first-generation flow cytometry (with two or three colors and high instrumental speed). The next challenge was distinguishing abnormal from normal developing clones after chemotherapy (hematogones), so more colors/markers were needed. The advent of modern cytometers allows gathering up to 6-8 markers in single staining, increasing the resolution power, and studying the co-expression of several molecules. Although classical microscopic techniques are still performed, MRD by molecular or cytometry techniques can redefine risk groups in complete morphological remissions19.
High sensitive molecular techniques have changed clinical outcomes, as documented by several controlled clinical trials demonstrating the superior prognostic value of MRD compared with leukocyte count, age, genotype, and early steroid response20. The purpose of MRD detection is to distinguish patients who respond well to therapy from those who require re-intensification, thus reducing high doses of chemotherapy in those patients at low risk, and recognizing patients who presumably have a low risk of relapse21. As previously mentioned, the prognostic value of MRD (currently determined by cytometry or RT-qPCR) is accepted as the most important factor in the clinical management of the disease due to a high correlation with the risk of early relapse22,23. Immunophenotyping at diagnosis is desirable but not necessary when following MRD. Innovative approaches used for tracking clones in B-ALL will be further discussed. However, such panels are not helpful for T-ALL and less so for myeloid origin leukemia, in which the recognition of the affected lineage at disease onset is essential for the appropriate choice of MRD markers. Some case reports describe leukemic reemergence with lineage changes, possibly due to leukemic subclones selection or the emergence of new clones during relapse24. Therefore, cytometry strategies should be sufficiently sensitive and phenotypically broad to identify re-emerging clones not associated with the lineage of origin.
Interestingly, MRD is also crucial in the prognosis of patients undergoing stem cell and hematopoietic progenitor transplantation, in whom MRD+ is often associated with unfavorable outcomes25.
Unfortunately, not all laboratories can determine MRD, as this does not depend on infrastructure but analysis and interpretation based on intensive training and in-depth knowledge of genotypic and phenotypic changes due to chemotherapy and pathways of differentiation of normal and malignant clones, among other biological aspects of the disease.
Technology applied to the diagnosis and monitoring of hematological neoplasms
The description of the double helix chain in the 1950s based on crystallographic studies by Rosalind Franklin was undoubtedly one of the most important discoveries for modern molecular biology. However, in the early 1960s, James Till and Ernest McCulloch demonstrated for the first time that bone marrow contained cells capable of regenerating blood in mice subjected to lethal doses of radiation. The hematopoietic system has been a gateway to several paradigms in medicine, as it has allowed various discoveries such as the description of the first cancer-associated translocation, the Philadelphia chromosome (by Janet Rowley). Later, this translocation led to discovering the first targeted therapy using inhibitors of the tyrosine kinase activity of the protein resulting from the fusion BCR-ABL and, of course, the discovery of cancer stem cells.
Undoubtedly, the development of monoclonal antibodies by Milstein and Köhler (1975) and the invention of flow cytometry made it possible to profile the immunophenotype of primitive cell populations and purify them through cell sorting. The development of these new tools prompted John Dick, who described a population parallel to that of normal stem cells capable of initiating leukemia. Research has expanded the knowledge of normal and pathological protein expression at the cellular level. The creation of consortia such as EuroFlow has allowed establishing the AL cell classification and detecting residual clones after chemotherapy.
The ability to amplify a specific region of the genome through PCR in the mid-1980s and, later, the ability to quantify the number of copies in real-time made it possible to measure, for the first time, the success of chemotherapy with molecular platforms (Fig. 2). Since then, and thanks to the human genome project, molecular tools such as massive NGS have made it possible to identify specific regions associated with cancer and new mutations, particularly those associated with leukemia. However, a combination of cellular and molecular strategies is recommended for the management of MRD18. Future technologies such as mass cytometry and other cytomics and molecular tools will allow highly sensitive detection of potentially MRD relapse-causing populations in the next generation.

Figure 2 Timeline of relevant discoveries for the biological characterization of leukemia. Todays technology has been tangible thanks to fundamental discoveries that allowed the birth of modern molecular biology and the consideration of leukemic stem cells as the functional origin of these diseases and, therefore, as a target for treatment.
Multiparametric flow cytometry
In daily practice, morphologic observations are usually confirmed by immunophenotyping. Although lymphoid blasts are usually distinguished from myeloid blasts, it is impossible to identify B or T lymphocyte blasts by simple colorimetric staining. Immunophenotyping is a highly specialized technique that uses flow cytometry to detect different fluorescent signals chemically coupled to antibodies that bind antigens with high specificity. As these antigens are usually membrane or cytoplasmic molecules, they can be detected simultaneously, if present in cells, according to the flow cytometers optical configuration. At present, immunophenotyping is based on panels of 6-8 colors (or more), translating into the detection of multiple fluorescences associated with different cell markers. At least in commercial panels, this information enables identifying the presence/absence of several key parameters for the classification of the most relevant hematopoietic lineages. Validated in vitro diagnostic (IVD) antibody panels are available commercially to confirm the clinical suspicion of AL through a cytometry approach. The EuroFlow Consortium has worked multicentrically to develop an intelligent combination screening tool that searches for eight specific markers of the B, T, and myeloid lineages, as well as molecules of hematological immaturity (Table 1). A correct analysis of such a guidance tube allows identifying AL of B, T, and myeloid lineage. Details on the aberrant expression of other molecules, identification of mixed phenotypes, and other markers of prognostic value are explored by adding other tubes with new antibody combinations.
Table 1 Antigens of the Euroflow panel ALOT (acute lymphoblastic orientation tube)
Antigen | Cells expressing the antigen |
---|---|
CD45 | Leukocytes |
CD34 | Stem cells and hematopoietic progenitors |
CD19 | B Lineage |
CyCD79a | |
smCD3 | T Lineage |
CyCD3 | |
CD7 | |
CyMPO | Myeloid |
The detection sensitivity for most commercially available flow cytometers is 0.01-0.001% (1×10−4-1×10−5), although the latter is not usually achieved. As the number of cells per sample is not always abundant, the acquisition of multiple tubes reduces the number of cells available for analysis. Therefore, the lower tubes acquired, the higher the number of markers involved (depending on the number of detectors of the equipment), and the higher the number of cells acquired, the higher the resolution capacity. Analysis software such as Infinicyt allows the fusion of tubes from four markersknown as the backboneand automatically calculates cell populations26. Achieving a high number of cells in samples obtained after chemotherapy is challenging because the collection of mononuclear cells may compromise the recovery of leukemic clones to the point of loss. Therefore, staining of the entire sample before bulk lysis is strongly recommended19.
Next-generation flow cytometry
Undoubtedly, one of the fundamental laboratory challenges for leukemia is the detection of MRD. Some protocols define MRD prognostic value as early as day 15, while others at day 28 or 33. The real challenge lies in finding residual clones with alterations in the normal maturation pattern or the presence of aberrant markers. Either scenario requires training in detecting normal cell maturation to identify pathological clones and markers to discern between developing cells and those that have survived chemotherapy.
As mentioned previously, flow cytometry can reach the detection limit of 1×10−5. However, to achieve maximum reliability, it is necessary to acquire an optimal number of cells (at least 5 million). There is no consensus on the minimum number of cells to define a cluster as a cell population; however, it is suggested to be between 10 and 50 events27. Another recommendation is to use specialized software for analysis, especially in MRD studies, where many nucleated cells (up to 10 million) have been acquired to search for residual clones.
Another challenge is the markers stability during treatment, as some disappear or are induced after rounds of chemotherapy28. Several studies have aimed to find the best combination of markers for disease tracing, especially in B-lymphoid origin leukemia, for which panels include, at least, the detection of CD19, CD79a, CD34, CD45, and CD3 antigens. Some options for monitoring MRD are further discussed (Table 2).
Table 2 Patients with positive blast populations for CD73, CD86, CD44, and CD304 antigens in various international studies
CD73
CD73 is an ectonucleotidase that produces adenosine (ADO) from extracellular ADO triphosphate (ATP). Under physiological conditions, it is expressed on the surface of some B cells and subpopulations of T and NK cells29. The role of CD73 is to create a suppressive environment after hydrolysis of extracellular ATP (damage signal) and reduce inflammation by generating ADO, a molecule with immunosuppressive potential. In a multicenter study, CD73 was shown to be aberrantly highly expressed on B-lineage blasts compared to its normal counterpart in 66% of the enrolled patients and was stable 15 days after initiation of treatment28. More recent reports have documented that CD73 has a greater tendency to increase significantly after treatment29.
CD304
CD304 (also called neuropilin 1) functions as a co-receptor for the vascular endothelial growth factor and semaphorin. It is expressed on plasmacytoid dendritic cells and some monocyte populations under physiological conditions, although normal pre-B populations usually express discrete levels30. Based on expression data and subsequently corroborating the findings by flow cytometry, Coustan-Smith et al. demonstrated the role of CD304 as a valuable molecule for monitoring MRD by comparing purified leukemic blasts with their normal counterpart4. B-ALL leukemic blasts significantly overexpress CD304. Its expression correlates with the presence of t(12;21) ETV6-RUNX1, which originates the TEL/AM1 fusion, and is inversely related to rearrangements in the MLL gene4,28,31. In combination with CD9, it could be helpful for the prediction of t(12;21) translocation32.
Interestingly, CD73 showed a positive correlation with CD304 expression, for which their combination results in a powerful strategy to identify residual clones during B-ALL (Fig. 3).

Figure 3 Differential expression of some markers useful for monitoring minimal/measurable residual disease. A. Differential expression of CD73/CD304 (left), CD66c/CD123 (center), and CD81 (right) in populations of residual B lymphoid blasts (CD45low CD34+ CD10+ CD20low), BII precursors (CD45low CD34+ CD38+ CD10+ CD20low/int), BIII (CD34- CD38+ CD10+ CD20low/int) and immature B cells (CD45hi CD34- CD38- CD10- CD20+). B. Dot plots of the different populations described above, the blasts population represents 0.0018% of the nucleated cells. APS: automatic population separator. Analysis performed in Infinicyt 2.0, Nereida Méndez.
CD86
Under physiological conditions, B cells express basal levels of CD86. However, after activation, its expression increases considerably. Besides, plasma cells maintain abundant expression. CD86 may be expressed on the surface of leukemic blasts in some patients with B-ALL, but there is no apparent correlation with any particular immunophenotype (pro-B or pre-B) or genetic subgroup. Rearrangements of the MLL gene and the BCR-ABL1 fusion protein have been associated with positivity to the marker. However, due to the low frequency of these subgroups, it is challenging to generate significant statistics28. The trend has been confirmed in at least half of the patients with B-ALL in whom CD86 expression has been explored, but few studies have addressed its potential use for MRD follow-up4,28.
CD58
Lymphocyte function-associated antigen 3 (LFA-3) or CD58 is expressed on the surface of antigen-presenting cells, especially macrophages. In most cases (> 90%), it is present in leukemic blasts and is stable in the treatment phase, making it a good candidate for monitoring MRD33. In a study that included 69 patients, CD58 helped detect ten patients with MRD (+) confirmed by PCR34. Despite being identified as one of the most outstanding molecules as MRD markers, other studies have shown that its implementation generates limited information for detecting remnant clones27,34.
CD66c
This glycoprotein participates in cell adhesion and is expressed on the surface of cells of myeloid origin under normal conditions; however, it is absent in non-pathologic lymphocyte populations. In B-ALL, this molecule is aberrantly expressed on the surface of blasts, especially in cases with the t(9;22) translocation that originates the BCR-ABL1 fusion protein35,36. Molecular subgroups with hyperdiploidy frequently express the CD66c antigen28,37. Recently, the EuroFlow Consortium observed that the combination of CD66c with CD123 provided valuable information (Fig. 3A and B) by the separation obtained of malignant populations27.
CD123
Myeloid progenitors, plasmacytoid dendritic cells, and basophils express the alpha chain of the IL-3 receptor (IL-3), CD123, whose signaling is involved in differentiation, proliferation, and survival processes. Experimental data confirm that CD123 is overexpressed on the surface of B-ALL and AML leukemic blasts; however, it is underrepresented in T-type leukemias38. Interestingly, the hyperdiploid genotype is associated with high CD123 expression. Therefore, in combination with CD86 and CD200, it could potentially identify this molecular subgroup4,39. In addition to being expressed in lymphoid cells, this molecule is currently used as a therapeutic target for immunological therapy since it is also explicitly expressed in leukemic stem cells of AML40.
CD44
CD44 is expressed in the cell membrane of various tissues and participates in cell adhesion and migration. During the early development of T cells, CD44 plays an essential role during the arrival of progenitors from the bone marrow to the thymus. Although its role seems more significant for T-ALL, B-lineage blasts express CD44 in almost 53.5% of cases4,30. Furthermore, its expression has been confirmed in AML blasts41. CD44 is one of the most studied tumor markers; its combination with CD24 has helped identify tumor stem cells in various solid tumors. Notably, BCR-ABL patients directly correlate with CD44 expression42.
CD81
CD81 belongs to a family of proteins known as tetraspanins. Under physiological conditions, CD81 is expressed in the surface of mature B and T cells, for which it is considered a molecule associated with lymphocyte maturation. Therefore, CD81 may be detectable from the early stages of lymphopoiesis, and its density increases as populations mature43-45. The absence of CD81 expression and positivity to other markers, such as CD58, marks suspiciously malignant populations44. Thus, the combination allows identifying residual blastic clones from developing normal progenitors (hematogones) (Fig. 3).
Mass cytometry
This technology merges two well-known approaches: flow cytometry and mass spectrometry. This combination generates proteomic analyses at a single-cell level. In practical terms, mass cytometry or cytometry by time-of-flight requires a suspension of cells labeled with antibodies coupled to pure elements (initially metals) instead of fluorochromes as in traditional flow cytometry. In this technique, cells are nebulized, and metals are associated with the cells and detected by mass spectrometry due to specific binding with the antibodies. Therefore, more than 40 proteins can be analyzed simultaneously without the need for compensation since each element is unique and identifiable by its atomic mass. Although this tool is mainly used in research, the number of parameters exceeds those achieved by the most equipped traditional cytometer. In the future, this technology could be applied to detect MRD with a much higher resolution46,47. However, one of the disadvantages is that the sample has to be disintegrated, so cells cannot be recovered for further studies. With the recent emergence of spectral flow cytometry, it is possible to investigate 48 colors/markers by analyzing the entire emission spectrum of each fluorochrome that is part of the panel rather than the peak emission, as occurs with conventional flow cytometry. Moreover, the development of cell purification kits based on this detection strategy is underway, as well as the detection of messenger RNAs useful for the most common genetic abnormalities (in combination with classical immunophenotyping). In the coming years, the implementation of specific oligonucleotide-conjugated antibodies will provide the possibility of single-cell transcriptomics based on traditional flow cytometry48.
Molecular biology in MRD
Before cytometry, MRD was traditionally measured through RT-PCR, detecting fusion products generated by translocations, although limited. With the development of digital PCR, a high precision quantification is possible now. Furthermore, the measurement of V(D)J rearrangement products allows identifying the immunological reconstitution of patients. However, as only one technique has been mainly used historically, few studies were initially consistent when combining techniques, possibly due to the lack of standardization49. Because of their predictive value, numerous technological and scientific efforts are underway to improve methods and innovate to identify clones that resist traditional therapy. Thus, results on massive sequencing techniques for MRD determination suggest a higher predictive accuracy for predicting relapse compared with other strategies50.
Recently, Waanders et al. demonstrated that relapse clones originated from subclones existing at diagnosis with variable abundance and identified some candidate genes that may undergo additional mutations such as NCOR2, USH2A, and NT5C2, evidenced by digital PCR before clinical relapse51. Undoubtedly, MRD detection is aided by cutting-edge technology but requires rapid and standardized techniques52. For low- and middle-income countries, the cost of such assays should be affordable for patients or public health services.
MRD in myeloid leukemia
Detection of gene fusion products by RT-qPCR is one of the most commonly used strategies in AML alongside flow cytometry. Since approximately 60% of myeloid leukemia belong to a molecular group, their traceability is facilitated by this method during treatment. At present, NGSs advantage is that it allows parallel information on the associated mutations and the detection of fusion products for these disorders53. Because of the high phenotypic heterogeneity within the same disease, there has been a significant delay in developing guidelines addressing all the characteristics. ALL protocols have been adapted to search residual cells through flow cytometry, but the results have differed considerably54. One of the biggest challenges for the cytometrist is distinguishing malignant clones from normal myeloid reconstitution. Despite the description of leukemic stem cells, only a few studies have followed these cells during MRD. However, given that the immunophenotype of CTLs can be variable between patients, proposing a panel that allows generalization is complex10. The search for stemness in AML during MRD and LICs in acute lymphoblastic leukemia would be handy.
MRD in the cerebrospinal fluid
Only about 30% of relapses occur in the central nervous system (CNS), and the detection of leukemic clones in the cerebrospinal fluid (CSF) at diagnosis occurs in < 15% of patients. These results are associated with a worse prognosis due to an increased risk of relapse55. The mechanisms underlying CNS colonization are not well understood, but experimental investigations indicate that blasts can cross the blood-brain barrier. Alternatively, potential seeding of blasts in the CNS during sample collection or prophylaxis protocols has been suggested56. Furthermore, the use of immunodeficient animal models has highlighted the determinant role of the antitumor surveillance system and the migratory capacity of leukemic populations in relapsing leukemia, distinguishable by a high expression of cortactin57.
Malignant blasts in the CSF are traditionally detected by cytology after concentrating the sample (cytospin). Moreover, blasts can be evaluated by flow cytometry using strategic combinations, including CD45, CD19, CD20, CD4, CD8, CD56, Ig lambda, Ig kappa, CD3, CD14, and CD38 antigens16, to identify the infiltrates cellular composition. A study of 673 patients showed that the relapse incidence at 4 years was higher in those where leukemic blasts were detected in the CSF by flow cytometry. Despite the superior advantages of flow cytometry over cytology, there is no consensus for leukemia diagnosis in the CSF, although several algorithms have already been thoroughly reviewed58. Other studies rely on molecular techniques for the detection of MRD in the CSF through PCR59. Regardless of the above, the biggest challenge is the correct preservation of the cellular material from the CSF, possibly implementing cell stabilizers for flow cytometry or nucleic acids. However, the low-volume sample cutting-edge techniques discussed here are also being explored for such frontier approaches60.
Future perspectives and challenges
MRD detection represents a challenge from several perspectives, including low cell number in aspirates, lack of smart marker configuration, need for cytometers with more than five colors, the involvement of highly trained analysts, and specialized software, among others. Sometimes, diagnostic immunophenotyping is not available, or the laboratories lack of systematization makes it challenging to follow-up residual clones. An advantage of hematological disorders with common molecular signatures is that such translocations can be monitored with high sensitivity by RT-qPCR. Moreover, mass cytometry or massive sequencing proposals will be explored in the coming years and addressed in pilot studies before being transferred to the clinic as routine protocols in our country. However, with the advancement of technology and the collaboration of different reference centers, most MRD measurement protocols are being standardized by conventional flow cytometry, which will provide greater accuracy and robustness. In Mexico, several initiatives for the consensus of markers and protocols for cytometry diagnosis will reduce the number of cases inaccurately diagnosed and evaluate treatment response and the increase in the overall survival of pediatric patients.