INTRODUCTION
Tumor cell lines have been widely used during decades to understand several aspects of tumor biology, including tumor initiation, progression, metastasis, and therapy response. Although easily available, immortalized commercial cancer cell lines face several disadvantages for individualized therapy since often they do not fully represent the molecular background of a patients tumor, and their long-term culture may lead to new genetic aberrations, thus limiting putative clinical correlations. These drawbacks underscore the need for patient-derived cancer cell models that better reflect the molecular characteristics of the original tumors and help design personalized, more efficient anti-cancer therapies. Figure 1 shows the general approach to use primary cancer cell cultures for personalized therapy. Generation of primary cancer cell cultures requires at least five stages that include ethical approval, biopsy acquisition, disaggregation, culture, and authentication.

Figure 1 Primary cancer cell culture for personalized therapy. Tumor tissue from cancer patients can be obtained by biopsy procedures such as endoscopy, needle aspiration, or surgery. Primary cell cultures can be developed using two-dimensional or three-dimensional culture systems, which are then employed to evaluate the response to specific anticancer drugs to improve prediction of patients therapy outcome. The approach harnesses drug testing on cancer cells with the same or highly similar molecular background as tumor cells in situ to help design a customized therapeutic strategy.
BIOPSY ACQUISITION AND SOURCES
Biopsies may be obtained by several methods. Endoscopic biopsy, for example, is done using a flexible tube (the endoscope) carrying attached tools for tissue collection as well as a light and a camera at the end to perform biopsies of bladder (cystoscopy), lung (bronchoscopy), and colon (colonoscopy) tumors, among others. Needle biopsy is used to obtain tumor cells from a suspicious lump. There are several variants of this procedure: (a) fine-needle aspiration, where a thin and long needle is inserted to reach the suspicious area (e.g., for thyroid nodules)1; (b) core-needle aspiration, which employs a large needle with a sharp tip at the end to extract a column of tissue; (c) vacuum-assisted biopsy, using a suction device to increase the amount of fluid or tissue obtained (e.g., for lung cancer)2; and (d) image-guided biopsy, aided by X-ray, computerized tomography, and ultrasound or magnetic resonance imaging to guide the needle acquiring the biopsy. If the sample cannot be taken by one of the previous methods, surgical biopsy is applied (e.g., breast lump, lymph node, and ovarian cancer)3. This method can be used to remove only part of the abnormal tissue (incisional biopsy) or the entire tumor (excisional biopsy). The sample should be procured under sterile conditions, placed into a tube containing media supplemented with fetal bovine serum (FBS) and antibiotics, and immediately delivered for processing.
Pleural or peritoneal effusions, ascites, or solid tumor tissues are the most common biopsy sources for primary cancer cell culture. Lung, breast cancer, lymphomas, and leukemia among other types of cancer are known to generate pleural effusions when tumor cells invade the pleura4. Ascites is common in ovarian, uterine, and cervical cancers5. Isolation of tumor cells from effusions or ascites is less complicated than from solid tumors coming from surgery. Usually, effusions are centrifuged; the supernatant is discarded and the cell pellet is resuspended in appropriate media supplemented with FBS, and the growth factors required according to the cancer type. The neoplastic origin can be characterized right after isolation. Pleural effusions are known to provide better efficiency at the establishment of primary cancer cell cultures6.
TUMOR DISAGGREGATION
Once the tumor biopsy has been acquired, the next step is to disaggregate tumor cells from the rest of non-malignant cells that include tumor-associated fibroblasts, immune cells, and blood vessels, among other extracellular matrix components7. Separation of tumor cells from the tumor microenvironment may be one of the main difficulties when establishing primary cancer cell cultures. Certainly, the right selection of the disruption method is needed to break down the extracellular matrix and the connective tissue architecture that surrounds cancer cells. Tumor tissue disruption into a single-cell suspension varies from manual homogenization to the use of automated dissociators. Mechanical, enzymatic, and chemical methods or their combinations are used to disaggregate tumor samples. Mechanical dissociation applies physical force to the tissue using a sharp blade, scissors, or simply scraping it on a plate surface. The tissue can also be passed through a steel or nylon mesh and aspirated repeatedly with a pipette or needle gauge8. Enzymatic dissociation uses a wide variety of enzymes such as proteases and collagenases that disaggregate the extracellular matrix9,10. Trypsin, elastase, pronase, hyaluronidases, and collagenases are the most common enzymes used for tissue disaggregation. Chemical dissociation disrupts Ca+2 and Mg+2-dependent cell junctions using chelators such as ethylenediaminetetraacetic acid or ethylene glycol tetraacetic acid that sequester cations to loosen intracellular junctions11. These three disaggregation methods have their own advantages and disadvantages (Table 1). There is also a semi-automatic tissue dissociator commercially available under the name gentleMACS (www.miltenyibiotec.com), featuring preloaded protocols to generate viable single-cell suspensions from tumor tissues12. Here, right calibration of concentration, time, and temperature is important to obtain good yield and cell viability. Depending on the tumor type, dissociation methods should be combined to improve the yield of primary cancer cells.
Table 1 Methods of tissue disaggregation
Types of tissue disaggregation | Advantages | Disadvantages |
---|---|---|
Mechanical | High yield Faster than enzymatic disaggregation |
Causes mechanical damage |
Enzymatic | Yields large numbers of cells with good viability
through optimization Faster than explants |
May affect cell membrane integrity if it is not well optimized |
Chemical Automatic dissociator | Maintains membrane integrity Easy disaggregation Safe and sterile handling High reproducibility |
Needs to be combined with another method
Expensive |
CULTURE AND MAINTENANCE OF PRIMARY CANCER CELLS
Primary cancer cells are becoming the gold standard as in vitro models in cancer research; therefore, their culture conditions should preserve the original tumor characteristics. Culture and maintenance of primary cancer cells are based on methods developed to grow immortalized cancer cell lines. Primary cancer cells can be grown by explants, two-dimensional (2D) or three-dimensional (3D) cultures, which will be described in the following paragraphs.
Culturing primary cancer cell cultures by explants has the advantage of retaining the tissue architecture and microenvironment, thus replicating, to some extent, the in vivo interactions. In this method, small tumor pieces are placed over a sticky surface that allows tumor to remain attached (Fig. 2a). Cells that outgrow the explant can be lifted off the plate and grown on 2D culture, and the explant can continue generating primary cancer cells13. Cancer cell cultures generated by explants are heterogeneous and might retain genetic variability for several passages. Explant cell cultures have been established from breast, prostate, and lung cancer14-16.
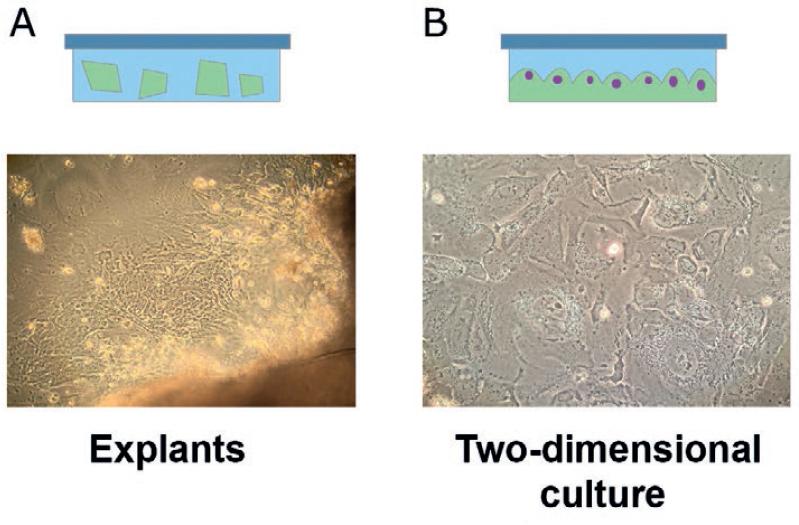
Figure 2 Schematic representation of growing primary tumor cells by explants and two-dimensional (2D) culture. (A) Tumor mass is cut into small pieces and placed on a sticky surface where tumor cells will outgrow from the explant. (B) Primary cancer cells grown in the 2D system.
Growing primary cancer cells by 2D cell cultures (2D) is based on the method used to grow immortalized cancer cell lines. This method was established more than 100 years ago, and at present, the culture conditions are well established. Primary cancer cell lines that have been disaggregated are grown on a flat surface using materials such as plastic or glass; sometimes, the surface is pre-coated with fibronectin, collagen, or synthetic extracellular matrix to improve adherence and spreading (Fig. 2b)17. The 2D cell culture system has the advantages of easy observation of the monolayer and manipulation of cells while being less expensive than 3D cultures. It has enabled countless breakthroughs in tumor cell biology research and has provided a vast amount of information useful for comparisons. However, the behavior of cells growing as a monolayer is different from that of the tissue in situ or even in 3D culture. Flat monolayers cannot reproduce the complex environment of in vivo tissue because interactions of surrounding cells are lost in three dimensions. Drug sensitivity may be increased since the surface in contact with the drug is larger on a spread cell18. For these reasons, results obtained with 2D cultures may not be entirely predictive for in vivo applications.
The third method for growing primary cancer cells is the 3D culture system, which in recent years has become a popular attempt to recapitulate in vivo conditions, including cellular interactions, specific environmental conditions (e.g., hypoxia, angiogenesis, necrosis, and metastasis), or presence of immune cells. In this sense, 3D cultures have proven to be more useful than 2D cultures for translational cancer research. There are different ways to culture cells as a 3D system, and they will be discussed below (Fig. 3).
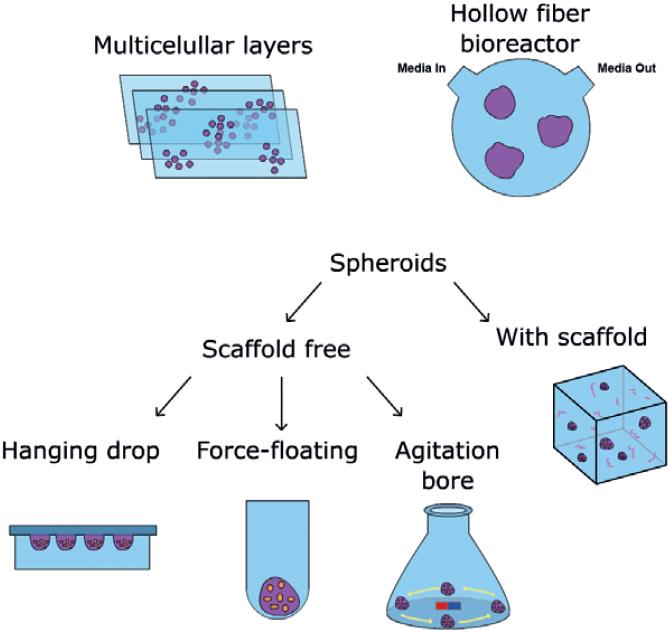
Figure 3 Schematic diagrams of three-dimensional (3D) primary cell cultures. Multicellular layers: primary cancer cells are embedded within membrane multilayers. Hollow fiber bioreactor is a continuous perfusion system that uses polyvinylidene fluoride fibers within a tube with an inlet where fresh medium flows, and an outlet where cellular products are excreted. Spheroids can be formed using a scaffold or scaffold-free system. Scaffolds are semi-solid matrices from synthetic or natural materials, for example, agarose, collagen, poly (ethylene glycol), and polyesters. The scaffold-free system does not require a solid support, and thus, spheroids are formed by placing cells in suspension (hanging drop), in a low adherent surface or continuous stirring to avoid cell attachment.
Multicellular layers
This method uses porous collagen-coated membranes where cells are placed. Then, several membrane sheets are put on top of each other. It is used with cells that do not aggregate, and its applications include measurement of invasion, drug transport, and resistance19.
Hollow-fiber bioreactor
3D culture by this method requires cells to be grown into polyvinylidene fluoride (PVDF) capillaries. It has been used to study cancer metabolism and drug resistance. Its main disadvantage comes from the barrier to cell growth that the PVDF fiber implies.
Spheroids
There are two types of spheroid culture systems: scaffold and scaffold free. In the latter, cell aggregates (spheroids) are generated without a solid support by one of the following methods: (a) the hanging drop, consisting of placing a drop of cell suspension on the underside of a cell culture plate lid to allow cell growth on the inverted lid20,21. Special hanging drop plates are available from companies such as NUNC and 3D Biometrix. (b) The force-floating method, where spheroids are generated by addition of a cell suspension to a low-adhesion plate after centrifugation; the plate is coated with hydrophilic or neutral charge polymer to avoid cell attachment22,23. (c) The agitation-based approaches, where spheroids are spontaneously formed using non-stop stirring. Cells in suspension are placed into a spinner flask with continuous high-speed stirring that prevents cells from attaching. Some advantages of this method are that spheroids are formed uniformly, throughput is high, and distribution of nutrients is homogeneous due to continuous agitation24.
The scaffold-based system for spheroid culture requires the use of extracellular matrix or a semi-solid organic or inorganic material where cells will survive, grow, and proliferate. This scaffold can be natural (agarose, collagen, fibronectin, laminin, or vitronectin) or synthetic (poly[ethylene glycol], poly[N-isopropylacrylamide] polyesters), and cells can just be put on the surface of the scaffold or embedded into it. Collagen type I is the most-used natural scaffold because of its low cost and pore size flexibility that accommodates different levels of matrix stiffness. Each type of cancer cell culture will require specific conditions; hence, concentration and scaffold type should be chosen carefully for optimal tumor cell growth. The scaffold system, however, presents some limitations regarding reproducibility and calibration of biochemical properties of the natural matrices; synthetic matrices can be more homogenous but present other constraints related to their opacity and limited capacity of penetration that hinders diffusion of testing compounds and further analyses.
Mimicking tumor formation in vitro through spheroid culture is widely used because it helps integrate various features of the tumor microenvironment. Coculture of tumor cells with fibroblasts, endothelial, or other type of cells can resemble the interactions occurring in vivo25,26. Spheroids can reach up to 1 mm in diameter; at this size, they have three regions. The center is a necrotic area due to lack of oxygen and nutrients; the middle area contains quiescent cells, and the outer layer has highly proliferating cells due to a great exposure to nutrients and oxygen. This structure is similar to in vivo conditions. Furthermore, cells may exhibit slower proliferation rates and increased survival when compared to 2D cultures. Molecular changes can also be found; for example, the ovarian cancer cell line SKOV3 grown in spheroids promotes expression of mesenchymal markers (N-cadherin, vimentin, and fibronectin) while diminishing expression of the epithelial marker CD32627,28. When malignant breast cancer cells are grown in laminin-rich extracellular matrix, apoptosis is increased and cell proliferation drops29. 3D cultures have also shown differences in drug response. Breast cancer cell lines grown in a laminin-rich extracellular matrix present resistance to anti-HER2 target drugs when compared to their 2D counterparts30.
PRIMARY CELL CULTURE CHARACTERIZATION AND AUTHENTICATION
Characterization and authentication are important steps after derivation of primary cancer cells. Characterization may include (a) demonstration of absence of cross-contamination; (b) confirmation of the species of origin; (c) connection with the tissue of origin, identification of the lineage to which the cell belongs and the differentiation state within the lineage; (d) determination of malignancy-associated characteristics; (e) predisposition of the new culture to genetic instability and phenotypic alteration; (f) identification of special features unique to the primary cancer cell culture; and (e) evaluation of growing parameters to determine optimal growth conditions.
Specific characterization techniques to be employed can fit into the type of work being carried out in the laboratory; for example, if molecular technology is easily available, then DNA fingerprinting, DNA profiling, or gene expression analysis is likely to be of most use, whereas a cytology laboratory may choose to use chromosome analysis coupled with fluorescence in situ hybridization and chromosome painting; a laboratory with immunological expertise may prefer to use major histocompatibility complex analysis linked with lineage-specific markers. These procedures in combination with functional assays should provide sufficient data to authenticate a primary cancer cell culture. Regardless of the intrinsic laboratory capacities, DNA fingerprinting or DNA profiling and multiple isoenzyme analyses have now become the major standard procedures for cell authentication. Either short tandem repeats31 or single-nucleotide polymorphism analyses best characterize human cell lines and can also be employed for primary cancer cells. Changes in morphology are frequently an early sign of deterioration and often indicate that cells are differentiating, contaminated, or experiencing a crisis. Nevertheless, there is the chance that a primary cancer culture could become cross-contaminated with an existing continuous cell line or misidentified by mislabeling or handling mistakes. Literature reports show that most cell lines in the United States in the late 1960s were contaminated with HeLa, a cell line derived from an invasive cervical carcinoma32-34. Characterization studies have become necessary for cell authentication and validation of the derived data.
Some valuable parameters of characterization for lineage and sublineage discrimination are (a) cell-surface antigens, which are particularly useful for hematopoietic cell sorting35. (b) Intermediate filament proteins - they are not involved in cell migration but appear to play a structural role and serve as lineage or tissue markers36-41. (c) Markers of differentiation and specialized function - these markers are unique and specific to the cell type; however, they depend on full expression of the differentiated phenotype. (d) Enzymes - constitutive enzyme levels (in the absence of inducers or repressors), induced or adaptive level (the response to inducers and repressors), and existence of isoenzyme polymorphism are useful parameters42. (e) Regulation - tumor cell microenvironment (matrix, adjacent cells) regulates the expression level of many differentiated products. Hence, determination of specific lineage markers may require pre-incubation of the cells in a suitable environment where hormones, growth factors, or extracellular matrix is present at the correct amount and concentration. (f) Lineage fidelity - lineage markers are frequently used as tissue or cell type markers; they are characteristic of cell function rather than its embryonic origin43,44.
BIOLOGICAL STUDIES USING PRIMARY CANCER CELL CULTURES
The use of primary cancer cell cultures for personalized therapy commonly resorts to assay cellular proliferation and migration as key biological properties to evaluate drug response.
Direct measure of DNA synthesis represents the most accurate way to assess cell proliferation. For example, 5-bromo-2-deoxyuridine (BrdU) is a reagent that incorporates into newly synthesized DNA during the S-phase of the cell cycle45. After denaturation, specific antibodies can detect the DNA-incorporated BrdU. A variation of this assay employs 5-ethynyl-2-deoxyuridine46. Cellular DNA content and metabolic activity represent indirect parameters to measure cell proliferation and viability. Fluorescent nucleic acid stains are commercially available for high-throughput screening in add-incubate-read workflow47,48. On the other hand, proliferation/viability assays based on cellular metabolism have the advantage of being more economical as compared with the previous methods49.
One common metabolic proliferation assay involves intracellular reduction of the tetrazolium salt 3-[4,5-dimethylthiazol-2-yl]-2,5-diphenyltetrazolium bromide by NAD(P)H-oxidoreductases of viable cells to form the formazan counterpart49-51. [3-(4,5-dimethylthiazol-2-yl)-5-(3-carboxymethoxyphenyl)-2-(4-sulfophenyl)-2H-tetrazolium] (MTS) can be employed in a similar fashion52. XTT [2,3-bis-(2-methoxy-4-nitro-5-sulfophenyl)-2H-tetrazolium-5-carboxanilide] and WST-8 [2-(2-methoxy-4-nitrophenyl)-3-(4-nitrophenyl)-5-(2,4-disulfophenyl)-2H-tetrazolium] are converted to soluble formazans in culture medium, but their reduction is thought to occur at the cell surface51. The soluble compound resazurin can be reduced into the fluorescent product resorufin and generally provides higher sensitivity than tetrazolium compounds49,53,54. Cell staining with crystal violet is a non-metabolic tool that can also be used to examine cellular proliferation55,56.
Cell migration and invasion are important traits that define primary cell phenotypes. Cell migration is of particular interest in cancer given that metastatic spread commonly represents an advanced stage in tumor progression and is strongly correlated with poor prognosis. One basic method to investigate cellular migration ability is known as the wound closure assay, which consists in the creation of a gap on a confluent cell monolayer to let cells on the edges move and close the wound57-59. The transwell migration assay, also known as the Boyden chamber assay, allows studying cellular migration through a physical barrier such as a porous membrane. Coating of the transwell membrane with extracellular matrix proteins turns the experiment into an invasion assay59.
PRIMARY CELL CULTURES FOR PERSONALIZED THERAPY
In the era of cutting-edge technology, enormous advances in cancer biology knowledge have led to a vast investment in drug discovery. However, these new target drugs have had limited clinical success due to high toxicity or ineffective response. For these reasons, the development of novel high-throughput screening models for testing new drugs in preclinical studies is vital to translate the results from bench to bedside. The methodology for anticancer drug screening has been developed since 1970s, and both in vitro and in vivo models have been used. For many years, tumor cell lines were the main models for drug screening; however, they present several caveats and poor clinical prediction power (Table 2). Other methods that better resemble a patients tumor have become more attractive and effective, such as patient-derived xenografts (PDXs), circulating tumor cells (CTCs), and primary cancer cell cultures. PDX is an in vivo model that retains most of the complexity of the original tumor, including its heterogeneity60,61. However, engraftment efficiency depends on the aggressiveness of tumor and can be influenced by the specific mouse model. The long time to develop the engraftment and cost-efficiency issues are some limitations of this model for drug screening and personalized therapy62-64 (Table 2). CTCs represent a metastatic stage and are suitable to study molecular changes developing from the primary tumor to the metastasis. At the moment, poor yield and purity are the main disadvantages of using CTCs for personalized therapy65 (Table 2). Primary cell cultures represent a very attractive ex vivo model for drug response prediction and informed clinical decision-making to improve therapeutic outcomes (Fig. 1). In recent years, their use has been expanding in preclinical studies with interesting results on intra-tumor heterogeneity and drug response from different tumor types. For example, Esparza-López et al. reported an association between cell and receptor tyrosine kinase expression patterns in subpopulations isolated from a primary breast cancer cell culture. Authors reported that platelet-derived growth factor receptor expression in breast cancer subpopulations drives cell proliferation, migration, anchorage-independent cell growth, and high sensitivity to paclitaxel and imatinib14. Kar et al. established several primary ovarian cancer cell cultures from ascitic fluid of corresponding patients66. After four to six passages, ovarian cancer cells were free of fibroblasts and were used to investigate the response to chemotherapeutic agents such as paclitaxel, carboplatin, and curcumin. Each primary culture showed a different percentage of apoptotic cells upon treatment with the single drugs; however, combination experiments demonstrated higher effectiveness. For example, the combination of carboplatin with paclitaxel increased the average percent apoptosis from 14% (individual treatments) to 22% and addition of curcumin increased apoptosis to 45%. Primary cultures uncovered the wide response variation to specific drugs, probably coming from distinct tumors molecular profiles. This highlights the potential of primary ovarian cell cultures for individualized therapy.
Table 2 Comparison of immortal cancer cell lines, primary cancer cells, PDXs, and CTCs for their use in personalized therapy
Cancer model | Advantages | Disadvantages |
---|---|---|
Immortal cancer cell lines | Standard culture media and techniques available | Prone to genetic and phenotypic changes over time |
Bypass ethical considerations on animal experimentation | Lack representation of tumor heterogeneity | |
Provide large number of cells useful for comparative studies | Lack relevance to personalized therapy | |
Suitable for genetic manipulation and molecular studies specific to cancer cells | ||
Lower cost | ||
Primary cancer cells | Reflect the molecular background of patients tumor cells | Cells may require complex culture medium to grow and retain phenotype |
Clinically relevant cancer cell models, useful for personalized therapy | Stromal components may outgrow cancer cells in the early culture steps | |
Bypass ethical considerations on animal experimentation | Tumor heterogeneity may be lost, depending on culture technique | |
Suitable for genetic manipulation and molecular studies specific to cancer cells | Generation efficiency depends on type and source of sample | |
Patient-derived xenografts (PDXs) | Resemble the parental tumor in vivo | Deficiency of host immune system |
Reflect the molecular background of a patients tumor including tumor heterogeneity | Engraftment efficiency may be biased to undifferentiated and more aggressive tumor subtypes | |
Clinically relevant cancer cell models, useful for personalized therapy | Mouse stroma outcompetes the human stromal component | |
Orthotopic xenografts resemble the growth and metastatic dissemination of a patients tumor | Development time may be too long for drug screening and personalized therapy Grafting techniques and mouse strains lack standardization Higher associated costs | |
Circulating tumor cells (CTCs) | Suitable to investigate the molecular profile of metastatic cancer, which could be different from the primary tumor | Current CTCs enrichment methods need improvement to attain better recovery and specificity rates |
Collected as liquid biopsy | Comprehensive molecular profiling is hindered by CTC yield and purity | |
Drug screening is challenged by low yield of CTC enrichment |
Another example of drug-response evaluation with primary cell cultures can be found in the work of Karekla et al.16. They developed a method for explant culture of non-small cell lung cancer (NSCLC) tumor samples retaining both tumor and stromal cells. Non-necrotic sections from fresh NSCLC tumors were identified by hematoxylin and eosin staining, and small tissue fragments were placed in microporous membrane inserts hanging in a well of a 6-well plate with culture medium. After 16-20 h, the inserts were transferred to new wells with fresh medium, and the explants were exposed to cisplatin and the targeted agent tumor necrosis factor-related apoptosis-inducing ligand (TRAIL) for 24 h. The explants were then fixed with paraformaldehyde and embedded into paraffin blocks to be analyzed by immunohistochemistry with cleaved poly-ADP-ribose polymerase (cPARP), Ki-67 and p53 antibodies. Previous experiments with a sample subset allowed the observation that significant drop in cell proliferation (Ki-67) and increase of cell death (cPARP) occurred with culture time beyond 24 h after the initial 16-20 h, whereas serum concentration in the growth medium had no significant effect.
The experiments exhibited the heterogeneous sensitivity of these preclinical models to the drugs and pointed out an enhanced prediction of chemotherapeutic benefit on a case-by-case basis. Only around 50% of explants responded to cisplatin treatment and most of them at high concentration (50 µmol/L). Notably, there was a significant correlation between patient survival and explant sensitivity to cisplatin in all tumor stages. In addition, percentage of cPARP staining showed dependency on histotype and was diminished in later tumor stages. Induction of p53 protein expression by cisplatin was associated with lower levels of cell death and suppression of cell proliferation. Treatment with TRAIL proved successful in one out of twelve samples. This work provides an advantageous approach in terms of time and costs over other methods such as patient-derived xenografts to gain information about NSCLC patient tumor response to anticancer drugs, enabling prediction of treatment response in a culture system that maintains the tumor microenvironment and takes advantage of immunohistochemistry for the specific analysis of tumor cells.
Recently, Kodack et al. reported the success rate of developing primary cancer cells from 568 patient tissues corresponding to a variety of tumor types and sources6. They found an average success rate of 26%, most of them being lung tumors. Pleural effusions had a higher rate (42%) compared to core biopsies (23%). The main cause for the lack of cell culture initiation was a low content of cancer cells in the patients specimens, followed by stromal fibroblast outgrowth. It was suggested that these difficulties could be alleviated by microscopic observation and differential trypsinization since fibroblasts tend to respond faster to cell detachment. Authors also found that a culture system with irradiated fibroblast feeder cells and medium with a defined composition (Dulbeccos Modified Eagles Medium, F-12, FBS, endothelial growth factor (EGF), insulin, adenine, hydrocortisone, cholera toxin, and Rho-associated protein kinase inhibitor) was more effective than standard growth media to generate primary cell lines (77% vs. 54% from needle biopsies). They went on to develop an immunofluorescence assay for cell viability applicable to biopsy cultures in the presence of stromal cells and found that cancer cells of epithelial origin can be identified by their expression of cytokeratin 8 (CK8) and cytokeratin 18 (CK18), including cells with epithelial to mesenchymal transition. CK8/CK18 could recognize NSCLC cancer cells, squamous cell lung cancer, breast, bladder, colorectal, and pancreatic cancer cells. The antibody pair did not stain human fibroblasts and could reproduce the doseresponse curves of pure cancer cells developed with an MTS-metabolic viability assay. Same results were found when cancer cells were cocultured with irradiated feeder fibroblasts. However, removal of EGF and insulin from the medium composition was needed to preserve the response to EGFR and ALK tyrosine kinase inhibitors, and this could slow down the establishment of the primary cultures. Sensitivity of the NSCLC biopsy cultures to tyrosine kinase inhibitors was found to be consistent with the patients response.
CONCLUSIONS
The fight against cancer has been moving toward personalized therapy in recent years. As we have discussed in this review, primary cancer cells represent an attractive model to study several aspects of cancer biology since they reflect the molecular and cellular characteristics of the original tumor cells. Culture of primary cancer cells involves a series of steps from ethics approval to sample collection, processing, maintenance, characterization, and authentication. Primary cancer cells are an excellent model for anticancer drug testing, overcoming deficiencies of cancer cell lines, or the costs associated with using animal models. As a valuable tool in personalized therapy, their use will likely keep expanding to improve prediction of cancer therapy benefit.