INTRODUCTION
The state of Hidalgo contributes to the national production with 0.5 % of Cu, 96.7 % of Mn, 2.7 % of Pb, and 2.8 % of Zn (SGM 2017). The municipality of Zimapán is a mining area representative of this state which produces undesired environmental impact of heavy metal in the residues and which could be of pronounced economic importance. This area presents a skarn of the metal type Zn-Pb-Ag-(Cu) in the form of sulfurous minerals including pyrite, arsenopyrite, sphalerite, galena, and others (Villaseñor-Cabral et al. 2000, Espinosa et al. 2009). Residues in these mines contains heterogeneous concentrations of arsenic (13 135 mg/kg), cadmium (610 mg/kg), copper (600 mg/kg), lead (3934 mg/kg), and zinc (11 363 mg/kg) (Armienta et al. 2016). After 70 years of accumulation of mine residues at this site, a significant volume has been generated and discharged in tailings ponds (Espinosa et al. 2009). If these residues get exposed to wind and rain the potential for dispersing and to contaminate the surroundings exist. Therefore, it is necessary to stabilize the mining residues for avoiding the chemical degradation of the environment.
In situ remediation techniques are utilized to stabilize mining residues, where the objective is not to change the total concentration of these metals, but to reduce the available fraction (Adriano et al. 2004). The most promising remediation techniques include the application of lime (Bolan et al. 2003), phosphates (Basta and McGowen 2004, Cui et al. 2016), biosolids (Wang et al. 2008, Placek et al. 2016), compost (Smith 2009), and more recently biochar amendments (Beesley et al. 2015, Mahar et al. 2015, Yuan et al. 2019). The application of lime initially increases pH, reduces the solubility of metals and can also mix with compost; in addition, is a low-cost material easily accessible and applied, but organic matter is transient reducing effectiveness afterwards (Gray et al. 2006, Kumpiene et al. 2008, Singh and Kalamdhad 2013). The application of phosphates forms stabilized precipitates of metal-phosphates and provides essential nutritional elements for the growth of the plant cover but they can cause leaching (Cao et al. 2009, Bolan et al. 2014, Osborne et al. 2015). Walker et al. (2004), and Singh and Agrawal (2008), have shown that the application of biosolids and compost decreases the bioavailability of metals, but their effect is variable depending on the metal, soil type, dose, and degree of organic matter humification. Most of these amendments require periodic applications and pre-treatments, which increase the application costs to ensure their success (Almas et al. 1999, Tandy et al. 2009, Cui et al. 2016, Gong et al. 2018). Biochar, on the other hand, is a solid product of biomass pyrolysis. It increases the recalcitrant organic carbon content of soil in the long term and requires a smaller number of applications compared to compost and biosolids. In addition, biochar is a porous material (Batista et al. 2018), presenting large specific surfaces for sorption of metals (Houben et al. 2013, Zhang et al. 2013, Wang et al. 2017, Wang et al. 2018, Yuan et al. 2019), improving soil physical properties (Tang et al. 2013, Bordoloi et al. 2019), and its pH value is normally around 5 to 12 (Yuan et al. 2019). Currently, there are studies where biochar has been modified by the addition of alkalis, oxidants (as O3, H2O2, K2MnO4 and air), microwaves, CO2 and steam to improve its sorption capacity (Zhang et al. 2016, Yuan et al. 2019). Nevertheless, it has positive and negative effects, depending on the method of activation, kind of bioassay and kind of soil (Koltowski et al. 2017).
The production and use of biochar from plants with high growth rates, present areas of opportunity to remediate acid residues generated by mining activity in Mexico. The water hyacinth has growth rates of 100-120 Mg/ha/year (Masto et al. 2013), besides its biomass possesses a strong adsorption capacity due to its high cellulose content and functional groups as carboxyl and hydroxyl (Patel 2012, Sindhu et al. 2017). For this reason, it has been used in wetlands, in solid dry form, like biochar, to remove toxic metals from aqueous solutions, wastewater and effluent treatments (Rezania et al. 2015, Sarkar et al. 2017, Neris et al. 2019).
Water hyacinth (Eichhornia crassipes Mart) is an invasive plant that for decades has affected the Endhó and Requena dams located in the municipalities of Tula, Alfajayucan, and Tepejí del Río, in the state of Hidalgo, México (Romero 1989, Soto 1989). It causes problems including an increase in sedimentation, canal blockages, invasion of water bodies, and competition with neighboring species, thereby decreasing biodiversity (Sindhu et al. 2017). This weed thrives in water bodies with high nutrient content and control can be manual, mechanic (by dredging or with a harvester machine), chemical (through herbicides), and biological (with herbivorous carps or insects). If this invasive plant is not removed from where it grows, it has the potential to recover with water availability. In addition, water hyacinth has large quantities of viable seeds that can germinate in the rainy season (Gutiérrez et al. 1994). The conversion of E. crassipes into biochar can represent a method for its management as weed and a use in the remediation of acid residues and soils contaminated with these, because it offers the possibility of neutralizing them and a greater permanence in the soil due to high resistance to microbial decomposition (Berek and Hue 2016, Li et al. 2016, Dai et al. 2017, Wang et al. 2018).
There are several studies that support the use of water hyacinth biochar as an amendment in soils or in mine residue’s remediation and polluted soils. In soils, its addiction increases the activity of active microbial biomass, soil respiration, the germination percentage and the shoots length of corn even with doses of 10 and 20 % (Masto et al. 2013); it also decreases cracking and increases water holding capacity when applied at 10 % (Bordoloi et al. 2019). For remediation it has an adsorption capacity for Zn and Pb of 22 and 45 % respectively, when is prepared with high pyrolysis temperature and at a 10 % dose (Wang et al. 2017); Cd is removed when is applied in a 2 % dose in a multimetal contaminated soil while As and Pb has little mobilization under acid precipitation (Yin et al. 2016). However, it has also shown the ability to remove aqueous anions such as phosphate (Cai et al. 2017) and arsenate (V) (Zhan et al. 2016) when it is magnetized by co-precipitation with Fe2+/Fe3+ on water hyacinth biomass before pyrolysis.
The objectives of this work were: a) to apply and evaluate biochar derived from water hyacinth (H) in mining acid residues; and b) to compare its performance with monopotassium phosphate (F = KH2PO4), lime [L = Ca(OH)2] and phosphate mixtures with biochar (FH) and lime (FL) by a bioassay of barley root growth, soluble metal and pH.
MATERIALS AND METHODS
Soils, mine residues and plant material
Acid residues (M4) were sampled from Dam # 5 of the Zimapán mining area, State of Hidalgo, Mexico (latitude 20º 43’ 58.1’’ N, longitude 99º 23’ 51.9’’ W). A pristine soil (M1), close to the zone of influence of the mine, was also obtained (see Guzmán 2012). These materials were air-dried, homogenized and sieved through a 2 mm mesh. Water hyacinth was collected from local dams on the Pátzcuaro lake, Uruapan, Michoacán (latitude 19º 34’ 7.21’’ N, longitude 101º 37’ 49.9” W).
Biochar production
Water hyacinth collected was air dried, milled and passed through a 3.5 mm mesh. Then it was slowly pyrolyzed in a modified Nabertherm oven at a temperature of 600 ºC, with temperature rise increases of 10 ºC/min, and a 30 min resilience time (Tang et al. 2013, Dai et al. 2017, Wang et al. 2017).
Neutralizing dose of mining residues
The amount of lime applied to the acid mining residues was determined by the titration curve method (Havlin et al. 1999, Aguirre 2001). A set of 13 threaded polyethylene bottle (50 mL) to which 10 g of the residues was previously added was prepared to receive increments of liming material [Ca(OH)2] at a rate of 1 mmol of OH- per reactor. The bottle one contained no lime. To each bottle, 30 mL of deionized water was added to reach a suspension ratio soil:water of 1:3 (w/v). The suspensions were shaken for 15 minutes and allowed to stand for 15 minutes before pH was measured. The first lecture was recorded as the pH at time zero. Subsequent pH lectures were taken every day during the first week and then every week until the readings stabilized and ceased to differ in value. The neutralization capacity was determined using AOAC 955.01 method (2005). The dosage of neutralization with biochar for the residues (M4) was evaluated following the procedure described above. To 11 threaded polyethylene bottles (50 mL) to which 10 g of residues were previously added (M4), 25 mL of deionized water and increments of biochar (starting with bottle two) at one percent per reactor (w/w). Neutralization-incubation (pH vs. mmol OH- or % biochar) graphs were constructed and neutralization kinetics curves (pH vs. time) drawn to determine the lime or the biochar necessary to reach pH stabilization time of the M4 mining residues. To calculate the need for lime (g/kg) to reach a pH of 6.5, the value of OH- mmol or biochar percent was interpolated from the graph. The pH 6.5 was considered optimal for plant growth and to immobilize metals and reduce their phytotoxic effect (USDA and NRCS 2000, Dai et al. 2017).
Substrates and treatments
A Cu, Pb and Zn metals concentration gradient consisting of four substrates of soil and mine residues was prepared to emulate natural degrees of pollution with mining residues: two mixtures of M1:M4 in percentage ratios (w:w) of 65:35 (M2) and 35:65 (M3); controls were 100 % pristine soil (M1) and 100 % acid mine residues (M4). Subsequently, the following amendments were added to the substrates: phosphates in the form of monopotassium phosphate (F), lime (L), water hyacinth biochar (H), and the mixture of monopotassium phosphate with lime (FL) and with biochar (FH). The M1 substrate did not include treatments L, and FL due to soil pH; each substrate served as a control, giving a total of 22 treatments. The dose of biochar added to the substrates was 10 % of their weight, following the suggestion made by Houben et al. (2013). The amount of phosphate used was based on a stoichiometric basis, that is, the amount applied corresponded to the sum of the study metals extracted with DTPA (diethylenetriamine pentaacetic acid). This was 6.2 g/kg of M4 and 0.6 g/kg of M1, according to Guzman’s previous works (2012). All treatments were incubated for 25 days in a solid:water ratio of 1:2.5 (w/v), without adding phosphates to avoid adsorption of this compound on soil particles. Because the doses of F and L were less than 1 % of the substrate weight, they were not considered for the calculation of the solid:water ratio of the incubations. After the incubation period was completed the substrates were air-dried and then the corresponding dose of phosphates was then applied to the treatments.
Physical and chemical analyses of biochar and treatments
The texture of M1 was determined by Bouyucos method and particle size distribution of the biochar by sieving (10, 30, 60 and 100 mm mesh). In control substrates and biochar-treatments the following parameters were measured: field capacity and permanent wilting point by the plate method and the pressure membrane (Klute 1986), respectively; the bulk density by the cylinder method (Blake and Hartge 1986a); the particle density by the pycnometer method (Blake and Hartge 1986b) and the saturation moisture in the saturation paste. In the control substrates and the biochar, pH and electrical conductivity (EC) were measured in water in a 1:20 solid:solution ratio, and according to the procedure of the International Biochar Initiative (IBI 2015); soluble concentration of Cu, Pb and Zn was determined in the same solid solution ratio as above; cation exchange capacity (CEC) was assayed by the silver thiourea (AgTU) method (Chhabra et al. 1975). Ag in the AgTU and the soluble metals (Cu, Pb, and Zn) were determined by atomic absorption spectrometry (Varian, Model Spectra AA55). The bases removed by AgTU solution, as well as Ca and Mg, and Na and K were determined by atomic absorption and atomic emission, respectively with a Varian 220 (Fast Sequential Model) equipment. The total metal concentrations in the control substrates and biochar were measured by X-ray fluorescence (Thermo Scientific NITON XL3T).
Assessing treatments
Due to the scarcity of normative risk values, especially for Cu and Zn, indicating whether a site should be treated to reduce the concentration of these metals and the mixture of different metals which can produce significant mixture effects, it is appropriate to use a bioassay with a plant sensitive to these metals (Baker and Walker 1989), as a diagnostic variable to evaluate phytotoxicity, once the remediation operations are carried out. This evaluation was based on a standardized root growth bioassay with a plant sensitive to heavy metals (Hordeum vulgare L. cv. Esperanza) and the correlation between pH and the extractable-soluble metal. Six barley seeds were planted at 3 cm deep in conical plastic vessels of 300 cm3 (ISO 1993) containing each treatment. Each assay was repeated three times. A bottom layer of sufficient inert porous volcanic material (size 4-8 mm) was applied to each vessel to allow drainage. The vessels were filled with 250 g of each treatment until 2 cm below the upper ring. The bioassay was carried out in a greenhouse. Daily watering was applied using 10 mL of deionized water per container. After emergence, the seedlings were allowed to grow for 24 days, and the roots of the plants were then extracted and measured. The experimental design was completely randomized. The mix pH and the metals solubility in water were measured by atomic absorption technique (IBI 2015).
Statistical analysis
Results were subjected to variance analysis (ANOVA) and the minimum significant difference (MSD p < 0.01) calculated. The pH, water-soluble metal and root length were correlated using the statistical program Minitab version 17.1.0. The level of significance used was 95 % unless otherwise stated.
RESULTS AND DISCUSSION
The texture of the soil (M1) was clayey and its pH-value, neutral, while mine residues (M4) sieved at 2 mm showed an acidic pH-value (Table I), while the pH-value of control substrates decreased below to M1. Acid mining residues added to the substrates caused an increase in acidity and salt content. These effects were attributed to in situ oxidation and residual sulfides (> 11 % acid drainage generators) in the mining waste (Guzman 2012, Labastida et al. 2013, Armienta et al. 2016). The electrical conductivity and the soluble Cu and Zn content, increased from M2 to M4. The removable bases, the CEC-values and the soluble Pb concentrations in the M2-substrate increased but in M3 decreased (Table I). Lead concentration in M4 was greater than 400 mg/kg, concentration that is above the standard of the Official Mexican Standard NOM-147-SEMARNAT/SSA1-2004 (SEMARNAT 2007), even though the soluble Pb content in all substrates was lower than the permissible limit of 5 mg/L of the Official Mexican Standard NOM-052-SEMARNAT-2005 (SEMARNAT 2006)., In Mexico does not exist a reference concentration considered dangerous for Cu and Zn. In contrast, the United States Environmental Protection Agency considers phytotoxic concentrations of 1500 and 2800 mg/kg for these metals (USEPA 1995). Only Cu was not toxic when increasing the percentage of mine acid residues in the substrates (Table I). When the percentage of soil was increased, the soluble concentration of these metals decreased due to the dissolution of carbonates present in the soil and mine residues (Labastida et al. 2013).
TABLE I MEAN VALUES AND STANDARD DEVIATIONS OF THE CHEMICAL PROPERTIES OF THE CONTROL SUBSTRATES AND BIOCHAR
Variable | Unit | M1 | M2 | M3 | M4 | H |
pH | 6.8±0.2 | 6.6±0.2 | 4.7±0.1 | 3.3±0.1 | 10.2±0.0 | |
EC1:20 | µS/m | 334.5±25 | 415±21 | 468±70 | 481.0±43 | 604±32 |
CEC | cmol(-)/kg | 27±3 | 46±1 | 24±6 | 22±5 | 42±2 |
RB | cmol(+)/kg | 39±1 | 50±1 | 21±0 | 18±1 | 424±3 |
CuT | mg/kg | 76±11 | 210±20 | 324±29 | 457±38 | 199±33 |
PbT | mg/kg | 173±3 | 2384±3 | 4279±3 | 6490±3 | < LD |
ZnT | mg/kg | 379±11 | 1090±18 | 1699±24 | 2409±31 | 115±20 |
Cu soluble | mg/kg | < LD | 0±0 | 1±0 | 11±0 | 1±0 |
mmol/kg | < LD | 5±1 | 10±1 | 169±4 | 19±1 | |
Pb soluble | mg/kg | 39±5 | 64±2 | 29±2 | 48±6.0 | < LD |
mmol/kg | 187±23 | 310±12 | 142±12 | 230±27 | < LD | |
Zn soluble | mg/kg | < LD | < LD | 5±0 | 29±2 | < LD |
mmol/kg | < LD | < LD | 82±0 | 449±35 | < LD |
LD = limit of detection for metals: total Cu 10 mg/kg, Pb 5 mg/kg and Zn 8 mg/kg; soluble Pb 1 mg/kg; Zn 0.1 mg/kg and Cu 1 mg/kg. Where: M1 is 100 % soil; M2 is 35:65 % (M1:M4); M3 is 65:35 % (M1:M4); M4 is 100 % acidic mining residues; EC1:20 is the electrical conductivity measured in a 1:20 ratio; CEC is the cation exchange capacity; RB are the removable bases; and CuT, PbT, and ZnT are the total metals
The application of H decreased the bulk density, the particle density (except for M3), the field capacity and the humidity at saturation (in M2, M3, and M4) and increased the pore space (Table II). Batista et al. (2018) determined that H (pyrolyzed at 350 ºC) had a high field capacity due to the porosity, the CEC, and the specific surface area. However, our results were opposite due to the pyrolysis temperature.
TABLE II AVERAGE VALUES OF PHYSICAL PROPERTIES OF CONTROL SUBSTRATES AND BIOCHAR SUBSTRATES
Substrate | Bulk density | Particle density | Field capacity | Permanent wilting point | Available humidity | Humidity saturation | Porous space* |
g/cm3 | cm3/cm3 | % | |||||
M1 | 1.18 b | 2.43 c | 0.43 a | 0.23 a | 0.20 ab | 0.72 a | 51.5 ef |
M1H | 0.85 e | 2.25 d | 0.39 ab | 0.20 b | 0.19 ab | 0.67 b | 62.0 a |
M2 | 1.13 bc | 2.43 c | 0.32 de | 0.14 c | 0.18 abc | 0.57 d | 53.2 de |
M2H | 0.97 d | 2.32 d | 0.38 bc | 0.17 c | 0.22 a | 0.60 c | 58.2 bc |
M3 | 1.29 a | 2.52 b | 0.29 ef | 0.15 c | 0.14 cd | 0.49 f | 48.8 f |
M3H | 1.08 c | 2.45 bc | 0.35 cd | 0.17 c | 0.18 abc | 0.61 c | 56.1 cd |
M4 | 1.34 a | 2.63 a | 0.21 g | 0.09 d | 0.12 d | 0.47 f | 49.2 f |
M4H | 0.99 d | 2.5 bc | 0.26 f | 0.10 d | 0.16 bcd | 0.53 e | 60.6 ab |
Different letters in the same column indicate significant differences (p < 0.05)
*Porous space was determined from the division between bulk density and particle density
Where: M1 is 100 % soil; M2 is 35:65 % (M1:M4); M3 is 65:35 % (M1:M4); M4 is 100 % acidic mining residues; and H is water hyacinth biochar
The CEC of biochar measured by AgTU-method is reported (Table I) without removal of carbonates and soluble salts. Singh et al. (2010) recommend measuring the CEC with the same method but with previous removal of salts, because Ag can precipitate with sulfides in pH > 8 and overestimate this measure. However, Doumer et al. (2016) and Batista et al. (2018) reported 37 cmol(-)/kg for H (pyrolyzed at 350 ºC) measured with ammonium acetate and barium acetate, a value close to the obtained in this work. The electrical conductivity and high alkaline pH value of the H was like those found by Singh et al. (2010), and Berek and Hue (2016).
The liming potential of the H was equivalent to 16.4 g/kg CaCO3. The equivalent dose necessary to correct the acidity of the mine residues and bring it to pH 6.5 was 5 % for H, as shown by the curves of neutralization of the biochars (Fig. 1A). This coincides with the dose found by Wang et al. (2017) who used water hyacinth (pyrolyzed at 500 ºC) to fix Zn and Pb while Houben et al. (2013) used 10 % (w/w) of Miscanthus giganteus biochar, pyrolyzed at 600 ºC, to reduce the activity of the metals from mining waste. The acid neutralization capacity of H can be attributed to lower aromaticity and a higher abundance of carboxylic groups (Doumer et al. 2016). Figure 1B shows a pH stabilization time for H of 6 days and the proportion of particles small than 0.148 mm of 30 %. Figure 2A shows the interpolated lime dose to correct the acidity of M4 up to pH 6.5, which was 9.2 mmol of OH- for 10 g of M4, equivalent to 34 g of Ca(OH)2/kg of the residues. The pH stabilization time was 34 days, a longer time in comparison to that required when biochar was used as a neutralizing agent (Fig. 2B). Biochar stabilized the pH of the residues in less time than lime. The differences in time of stabilization of the M4 pH when using the biochar can be attributed to multiple factors, such as the type of biomass, the particle size and the dose, and the pyrolysis temperature, among others (Tang et al. 2013, Zaccheo et al. 2014, Wang et al. 2017). Jones et al. (2012) indicated that the neutralization potential of a wood mixture biochar (pyrolyzed at 450 ºC), was suppressed after three years of being added to the soil, mainly due to the leaching and loss of alkaline elements. Similarly, Ruttens et al. (2010) reported that over time calcareous amendments can be dissolved and leached in the soil. On the other hand, Wong et al. (1998) showed that mineral residues with pH < 3 had no potential acidity formation due to the high content of oxidized sulfurous minerals. In our case, the potential acidification value of the acidic mineral residues was zero (Cruz R. E. pers. comm. 2016), therefore the loss of liming material rarely occurs.
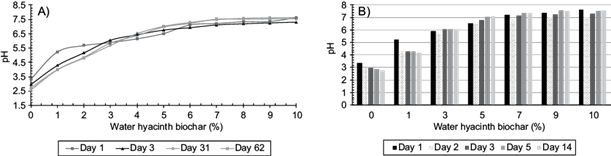
Fig. 1 (A) Neutralization curves (pH vs % biochar) and (B) kinetic effect of different application rates of water hyacinth biochar to the acidic mine residues (% w/w)

Fig. 2 (A) Neutralization curves (pH vs. mmol OH- lime) and (B) kinetic effect of different application rates of lime to the mine residues (mmoles OH- in 10 g)
In order to define a critical reference level (p < 0.01) for root growth and use it as an indicator of phytotoxicity and treatment efficacy, the mean root length of the pristine soil (M1) was employed, this was considered to be the minimum mitigation value to be achieved. All treatments raised the pH to the M1 with the exception of M2F, M3, M3F, M4 and M4F. The root length was increased in all treatments except for M4 and M4F due to the high phytotoxic chemical activities of the H+ ions and the high levels of soluble Cu and Zn. This high concentration of H+ ions is caused by the hydrolytic oxidation of minerals such as pyrite (FeS2), which causes the release of free sulfuric acid (Tordoff et al. 2000). The soluble Pb was increased in M2 and M2F above M1 (Fig. 3).

Fig. 3 Effect of treatments on barley root length (A), pH (B), soluble copper (C), lead (D) and zinc (E) on substrates soil-acid mining residues gradient. Different lower case letters of each bar indicate statistical differences (Fisher test, p < 0.01). Where the first letter and the subscript indicate the substrate material employed (M1 is 100 % soil; M2 is 35:65 % (M1:M4); M3 is 65:35 % (M1:M4); and M4 is 100 % acidic mining residues) and the last letter(s) indicate the applied treatment, where F corresponds to monopotassium phosphates, L to lime, H to the water hyacinth biochar
Addition of H, FH and FL treatments to M4-substrate increased the root length and reduced the soluble Cu and Zn compared to the control (M4H, M4FL, M4FH > M1, M4L > M4F, M4) (see Fig. 3). Response of these treatments was equal to or greater than that of M1, due on the one hand to the increase in pH, which favored the decrease in the chemical activity of H+ ions and the phytoxicity caused mainly by the sorption of metals in the solid phase (Dai et al. 2017).
The presence of soil in 2/3 and 1/3 parts for M3 and M2 substrates was enough to mitigate the phytotoxic effect because results of root growth were equal to the treatments. For M3-substrate all treatments showed performances above the pristine soil reference level (M3, M3L, M3F, M3H, M3FL > M3FH > M1) while in M2-substrate only the control (M2, M2L, M2F, M2FL > M2H, M2FH, M1). According to figure 3, in M1 no soluble Cu was detected, but was present in the treatments of substrate (M3), supporting the positive contribution of this metal to plant response. Lime reduced soluble Pb (M3L and M2L) and soluble Zn were reduced with L, FL, H and FH. Table III shows the correlation coefficient between the pH and the concentration of the soluble metal, and between the soluble metal and the root length. The negative correlation coefficients are related to the increase in extractability of the soluble metal at low pH-values (less than 4), while reducing root growth. These relationships are indicative of phytotoxicity/phytoavailability levels of the Cu and Zn in the treatments studied. The values of the correlation coefficients do not allow to conclude that the increase of root length for substrate M3 was mainly due to the decrease in the phytotoxicity of soluble Cu, but to be phytoavailability.
TABLE III CORRELATION COEFFICIENT AND P-VALUES IN pH-SOLUBLE METAL AND SOLUBLE METAL-ROOT LENGTH
Metal | pH-soluble metal | Soluble metal-root length | ||
Correlation coefficient | p-value | Correlation coefficient | p-value | |
Cu | -0.682 | <0.001 | -0.641 | <0.001 |
Pb | -0.272 | 0.146 | -0.070 | 0.071 |
Zn | -0.790 | <0.001 | -0.724 | <0.001 |
For the M2-substrate, the H and FH treatments generated less growth of the root compared to M2. The pH of treatments with neutralizing power (L, H, FL and FH) was higher than 7 and the concentration of Pb was also lower than when lime was added (pH = 5.9); Zn was not detected in all treatments of this substrate. The increase in root length promoted by the different treatments of substrates M2 and M3 relative to the reference materials M1 and M4 was expected because on one hand, soils, and treatments with biochars (Lu et al. 2015, Jiang et al. 2016, Wang et al. 2017), lime (Bolan et al. 2003) and phosphate (Cao et al. 2009, Osborne et al. 2015) naturally tend to reduce mobility and mitigate at some degree the phytotoxicity of heavy metals such as Cu, Pb, and Zn. Adriano et al. (2004)) reported that when the amendment was applied new free organic and inorganic functional groups are available for tfor the complexation and sorption of metals, which would explain the higher root length.
On M1-substrate, the application of phosphates promoted the root growth when compared with the rest of the treatments (M1F>M1,M1H, M1FH), and the treatments with H and FH were not significantly different to M1 (M1F > M1, M1H, M1FH). Karami et al. (2011) proved that wood biochar applied at a dose of 20 % (v/v) reduced the availability of phosphorus and Mosa et al. (2018) reported phosphate sorption on water hyacinth 3biochar. When phosphate was applied together with H to the substrates, produced the same effect on growth root and soluble Cu and Zn, that when they were applied for separately. The value of pH was greater with H than with FH in M2, M3 y M4, whereas the soluble Pb content was 60 % and 88 % lower with H and with L, respectively, for the substrate M2.
CONCLUSION
Water hyacinth biochar showed to be an effective amendment to reduce the solubility of Cu and Zn and to neutralize the pH in less time than limeand it also increased the porous space of the mining residues. The reduction of soluble Pb with lime was greater than biochar in the mixtures soil:residues. However, the biochar, as the other amendments, caused root length increase when the residues were above of 65 % (M3 to M4). Its effects on pH and soluble Cu and Zn were comparable with lime, phosphate-lime and phosphate-biochar. When water hyacinth biochar was applied to pristine soil its effect on the evaluated variables was not evident.