1. Introduction
Chromitites chiefly consist of Cr- and Al-rich chromite (generally, >80 vol.% of chromite; Arai and Miura, 2016), which represent the main reservoir of chromium in nature. Chromitite orebodies large enough for mining are spatially associated with mafic and ultramafic rocks and their serpentinized equivalents, and are generally found in: i) continental layered intrusions forming stratiform chromitites, and ii) different parts of the oceanic ophiolitic sequences as a “podiform” or “stratiform” chromitites (e.g., Leblanc and Nicolas, 1992; Stowe, 1994; González-Jiménez et al., 2014 and references therein). Besides their economic importance, chromitites are also scientifically relevant because the composition and microstructural features of chromite, and the mineralogy of their inclusions (i.e., ultra-high pressure, super-reducing and continental crust minerals) provide valuable information about the nature, evolution and geodynamics of the Earth’s lithosphere (e.g., Dick and Bullen, 1984; Arai, 1992; Barnes and Roeder, 2001; Kamenetsky et al. 2001; Rollinson, 2008; González-Jiménez et al., 2014; Arai and Miura, 2016; Griffin et al., 2016; Xiong et al. 2017; Farré-de-Pablo et al., 2019; Pujol-Solà et al., 2018; Tanner et al., 2019).
Mineralogical, geochemical and thermodynamic studies performed in “meta-chromitite bodies” from suprasubduction zone ophiolitic sections in Tehuitzingo and Loma Baya in southern Mexico (Proenza et al., 2004; Ortiz-Hernández et al., 2006; González-Mancera et al., 2009; González-Jiménez et al., 2015, 2017; Colás et al., 2019; Farré-de-Pablo et al., 2019), Tapo in Peru (Castroviejo et al., 2009; Tassinari et al., 2011; Colás et al., 2017), and La Cabaña in Chile (Barra et al., 2014; González-Jiménez et al., 2016; Plissart et al., 2019) suggest that during their exhumation the primary silicates (olivine and pyroxene) are transformed by serpentine and chlorite, while the magmatic chromites are replaced by Fe2+-rich porous and/or Fe3+-rich non-porous chromite. This metamorphic alteration takes place in presence of reducing/oxidizing (Fe3+-) SiO2-rich and MgO-poor fluids, at temperatures below 700 ºC (e.g., Gervilla et al., 2012; Barra et al., 2014; Colás et al., 2014, 2017, 2019; González-Jiménez et al., 2016, 2018; Farré-de-Pablo et al., 2019). In contrast, the dehydration of previously hydrated chromitites by contact metamorphism turns back the metamorphic reaction, forming a non-porous chromite with a composition close to primary chromite and Mg-rich olivine, at temperatures above 550-600 ºC (González-Jiménez et al., 2015). The analysis of chromite using LA-ICP-MS have revealed that during metamorphism the cores of chromite grains may retain their initial magmatic signature, particularly during the formation of Fe2+-rich porous chromite (Barra et al., 2014; Colás et al., 2014, 2017, 2019; González-Jiménez et al., 2015, 2016; Kapsiotis et al., 2018; Yu et al., 2019). Meanwhile, several minor and trace elements (i.e., V, Sc, Ga, Ti, Ni, Zn, Co and Mn) may pervade into the cores during the formation of Fe3+-rich non-porous chromite (González-Jiménez et al., 2016, Colás et al., 2019). This demonstrates that minor and trace elements are mobile during the alteration of chromitite bodies and, therefore, they define fingerprints of retrograde hydrous metamorphism. However, this issue has never been examined on chromitites affected by prograde regional metamorphism.
In this research work we provide a detailed study including petrographic observations, analysis of major, minor and trace element composition of chromites, coupled with thermodynamic modelling for chromitite bodies from Los Congos and Los Guanacos ultramafic massifs in the Eastern Pampean Ranges, northern Argentina (Escayola et al., 2004; Proenza et al., 2008). These ultramafic massifs are among the few known examples of suprasubduction zone ophiolites in Latin America that contain a mantle section with chromitites that have experienced high-temperature metamorphism as a result of a continent-continent collisional orogeny (e.g., Escayola et al., 2007; Collo et al., 2009). Therefore, this regional context is important to evaluate the geochemical fingerprints of prograde metamorphism in chromites from ophiolitic chromitites.
2. Geological setting
The Eastern Pampean Ranges constitute the easternmost exposure of the metamorphic basement of the Central Andes, in northern Argentina (Figure 1), and represent an accretionary orogen developed during the Neoproterozoic-Early Cambrian at the SW paleo-Pacific margin of Gondwana (e.g., Escayola et al., 2007; Collo et al., 2009). The Eastern Pampean Ranges are mainly composed of low- to high-grade metamorphic sedimentary and igneous units that are essentially constituted by garnet-biotite gneiss and biotite schists, with minor occurrence of marbles, phyllites, amphibolites and ultramafic rocks of ophiolitic affinity (e.g., Escayola et al., 1996, 2004, 2007; Rapela et al., 1998, 2016). These units were locally migmatized (i.e., San Carlos Massif and Yacanto Group; Martino et al., 1995; Siegesmund et al., 2010; Guereschi and Martino, 2008) and intruded by Early Cambrian granitoids (Rapela et al., 1998; Escayola et al., 2007) (Figure 1). All the basement units were intruded by Ordovician and Devonian metaluminous to peraluminous granitoids (e.g., Rapela et al., 1998, 2018; Pinotti et al., 2002; Geuna et al., 2008) and were locally covered by Cretaceous volcanic-sedimentary sequences and Cenozoic volcanic rocks (Ramos et al., 1991; Kay and Gordillo, 1994; Lagorio et al., 2016 and references therein) (Figure 1).

Figure 1 Simplified geological map of the Eastern Pampean Ranges (modified from Martino, 2003 and Siegesmund et al., 2010) showing the location of Los Congos and Los Guanacos ultramafic massifs hosting the chromitite bodies investigated in this work.
The ultramafic bodies are arranged into two NW-SE belts associated with ductile shear zones that crosscut the migmatitic massifs, gneisses and schists (Figure 1). Los Congos and Los Guanacos ultramafic massifs (up to 1.3 km long and 0.7 km wide) are merely 6 km away of each other, and outcrop in the southwestern ophiolitic belt of the Eastern Pampean Ranges, along the Sierra de Comechingones (Figures 1 and 2). Such massifs consist of partly to strongly serpentinized harzburgites that host chromitite bodies, which are accompanied by lenses of dunites and impregnated peridotites, and are cut by pyroxenite, gabbro and basalt dikes (0.5 to 1 m thick and several meters long) in Los Guanacos massif (Escayola et al., 2004, 2007; Proenza et al., 2008). These lithologies are in tectonic contact with metapelites, tonalitic gneisses and marbles (Figure 2), and were considered as a fragment of a Neoproterozoic (647 ± 77 Ma; Escayola et al., 2007) back-arc ophiolite sequence represented by: i) the porphyroclastic harzburgite tectonites in Los Congos massif, and ii) the Moho Transition Zone (MTZ) in Los Guanacos massif (Escayola et al., 2004, 2007; Ramos et al., 2010).

Figure 2 Simplified geological map of Los Congos and Los Guanacos ultramafic massifs (modified from Escayola et al., 2004).
Based on mineral assemblages and textures, Escayola et al. (1996, 2004) distinguished four metamorphic events in the ultramafic rocks of the Sierra de Comechingones. The first event of metamorphism occurred at ocean floor conditions, and is characterized by the replacement of primary olivine by lizardite/chrysotile with mesh and pseudomorphic textures, and by the replacement of pyroxene by bastite (<300 ºC and <4 kbar). During the second event, the regional prograde metamorphism produced the transformation of lizardite to antigorite along with olivine-bearing assemblages at 780-800 ºC and <10 kbar. The third event of metamorphism led to the retrogression of olivine to anthophyllite and antigorite+brucite at 500 ºC. The last metamorphic event is related to the formation of veins of talc+chrysotile during a low-temperature hydrothermal event. The second and the third metamorphic events recorded in the ultramafic rocks were associated with the collisional episode and the subsequent decompression and exhumation of the accretionary orogeny.
These metamorphic events were also recorded in the basement of Eastern Pampean Ranges. The second stage of metamorphism occurred under granulite facies conditions, peaking at 800 ± 150 ºC and 7.5 ± 5 kbar, and the retrogression to the amphibolite facies occurred at 600 ºC and 4.5 ± 1.5 kbar in gneisses, migmatites and granulites from the Sierra de Comechingones (Otamendi et al., 1999, 2004, 2005). However, higher pressure metamorphic peak conditions (810 ± 50 °C and 8.6 ± 0.8 kbar), and the highest temperature for the amphibolite facies overprint (715 ± 15 °C and 4 ± 0.5 kbar) were estimated in metagabbros, garnet migmatites and tonalities from the Sierra Chica and Sierra Grande (Rapela et al., 1998). In addition, Martino et al. (2010) reported similar metamorphic peak conditions (804 ± 36 ºC and 7.9 ± 0.7 kbar) on garnet gneisses of the Sierra Chica, but recorded a lower temperature and higher pressure overprint (649 ± 61 ºC and 6.2 ± 1 kbar) than Rapela et al. (1998).
The chromitite samples collected for this study were obtained from six chromitite bodies from Los Congos and Los Guanacos ultramafic massifs (Proenza et al., 2008). Chromitite bodies occurred as small pods and layers, few tens of meters long and less than 5 m thick, and are enveloped by dunites of variable thickness, enclosed in metaharzburgites, and occasionally crosscut by pyroxenite, gabbro and dolerite dykes (Escayola et al. 2004; Proenza et al., 2008).
3. Analytical methods
Selected chromite grains that preserved the textural varieties described by Colás et al. (2016) were imaged using a scanning electron microscope (SEM) model JEOL SM 6400 SEM equipped with energy-dispersive spectrometry and back-scattered electron (BSE) detectors available at the Universidad de Zaragoza (Spain). Additional BSE images of chromites were performed with a Swift-ED Hitachi TM-1000 Environmental Scanning Electron Microscope (ESEM) available at the Laboratorio de Petrografía y Microtermometría of the Instituto de Geofísica, Universidad Nacional Autónoma de México (Mexico).
Major-element composition of the different phases in the textural varieties of chromite has been analysed using a CAMECA SX-100 electron microprobe (EMPA) available at the Geochemical Analysis Unit of the CCFS/GEMOC, Macquarie University (Australia) under the following working conditions: 20 kV acceleration voltage, 20 nA beam current and 2-3 µm beam size. Peak counting times were 10 s for Cr, Fe, Ti, V, Mn, Zn, and Ni, 20 s for Mg, and 30 s for Al. Natural and synthetic standards used were MgO (Mg), olivine (Si), kyanite (Al), sphalerite (Zn), magnetite (Fe), rhodonite (Mn), rutile (Ti) and pure metals for Ni, Cr and V. Matrix correction were applied followed the method by Pouchou and Pichoir (1991). Additional chromite analyses were performed using a CAMECA SX-50 EMPA instrument belonging to the Centres Científics i Tecnològics of the Universitat de Barcelona (CCiTUB, Spain). The analytical conditions were 20 kV accelerating voltage, 20 nÅ beam current, 3 μm beam diameter, and counting time of 20 s for Mg, Al, Si, Ti, and Cr, and 30 s for V, Mn, Fe, Ni, Zn and Co. Calibration standards were natural and synthetic materials: periclase (Mg), corundum (Al), diopside (Si), rutile (Ti), Cr2O3 (Cr), rhodonite (Mn), magnetite (Fe), NiO (Ni), sphalerite (Zn) and pure metals for V and Co. The correction procedure XPP (Pouchou and Pichoir, 1991) was used to convert specimen intensity ratios into concentrations. The chemical data for chromite were stoichiometrically recalculated in order to distinguish FeO from Fe2O3 according to the procedure described by Droop (1987).
The quantitative analyses of silicates were carried out using a JEOL JXA-8230 EMPA instrument available at the CCiTUB (Spain) under the following working conditions: 20 kV acceleration voltage, 20 nA beam current, 2 µm beam diameter and counting time of 10 s per element. The standards used are albite (Na), periclase (Mg), corundum (Al), diopside (Si), wollastonite (Ca), rutile (Ti), Cr2O3 (Cr), rhodonite (Mn), magnetite (Fe), and sphalerite (Zn). The correction procedure XPP (Pouchou and Pichoir, 1991) was used to convert specimen intensity ratios into concentrations. Additional silicates were analyzed using a JEOL JXA-8900R EPMA instrument available at the Laboratorio Universitario de Petrología of the Instituto de Geofísica, Universidad Nacional Autónoma de México (Mexico). The analytical conditions were 20 kV accelerating voltage, 20 nÅ beam current, and 1 μm beam diameter. Peak counting times were 40 s for Al, Ca, Cr, Fe, Mg, Mn, Ni, Si and Ti, and 10 s for Na and K. ZAF corrections were applied online. The calibration was performed using natural and synthetic standards: albite (Na), diopside (Mg), kaesurtite (Al), diopside (Si, Ca and Cr), ilmenite (Ti and Fe), olivine (Ni and Mn). All EMPA data of chromite and silicates are listed in Appendices 1 and 2, respectively.
Minor- and trace-element compositions of the different phases in chromite grains were analysed using a New Wave UP 266 laser system connected to an Agilent 7500cs ICP-MS available at the Geochemical Analysis Unit of the CCFS/GEMOC, Macquarie University (Australia), following the method described by Colás et al. (2016). For this study, the LA-ICP-MS analyses of chromite were focused on the 45Sc, 47Ti, 51V, 55Mn, 59Co, 60Ni, 66Zn and 69Ga masses, and are listed in Appendix 3.
The thermodynamic modelling of phase relations during the alteration of chromite was performed using the Perple_X 6.8.3 software (Connolly, 2009), and the internally consistent thermodynamic database extended to include Cr-bearing phases (Holland and Powell, 1998, revised in 2002; cr_hp02ver.dat). The components considered to perform the pseudosection calculations of chromitite sample were computed in the close system FeO-Fe2O3-Cr2O3-MgO-Al2O3-CaO-SiO2-H2O (FCrMACaSH), and the solid solutions were Cr-spinel (Colás et al., 2019), antigorite (Padrón-Navarta et al., 2013), magnetite (Sack and Ghiorso, 1991b), olivine, chlorite, orthopyroxene and clinopyroxene (Holland and Powell, 1998). All other minerals involved in the calculations are pure phases. The Fe2O3 component in the textural varieties of chromite was considered as the magnetite solid solution phases, while the Cr-bearing chlorite was not considered because of the lack of experimental data on the mixing parameters for Al and Cr in this mineral.
4. Petrography and chemistry of chromitite orebodies
4.1. Chromite
The chromitite bodies consist of massive (>80 vol.% chromite), semi-massive (50-80 vol.% chromite), and to a lesser extent, disseminated (30-50 vol.% chromite) textures. These chromitites were intensely modified by metamorphism, which has produced the three textural varieties of chromites defined by Colás et al. (2016) (Table 1; Figure 3). Type I chromite, without exsolved phases and optically homogeneous (Figure 3a), shows a trend of increase of Cr# [Cr/(Cr+Al) atomic ratio] coeval with a decrease of Mg# [Mg/(Mg+Fe2+) atomic ratio] displaced at lower Mg# from massive (Mg# = 0.52-0.68 and Cr# = 0.47-0.72), semi-massive (Mg# = 0.48-0.55 and Cr# = 0.60-0.73) to disseminated (Mg# = 0.42-0.52 and Cr# = 0.55-0.63) chromitites (Table 1; Figure 4a to 4c). The Fe3+/(Fe3++Fe2+) ratio varies from 0.43 to 0.54 in Type I chromite from massive and disseminated chromitites, but is lower in Type I chromite from semi-massive chromitites (Fe3+/(Fe3++Fe2+) = 0.24-0.27) (Table 1; Figure 4a to 4c). Type II chromite shows a magnetite-rich phase (Mg# = 0.21-0.23, Cr# = 0.85-0.87, and Fe3+/(Fe3++Fe2+) = 0.62-0.63) that forms irregular blebs randomly distributed along the rims or linear strings, and fine lamellae with apparent crystallographic control hosted in a spinel-rich phase (Mg# = 0.56-0.63, Cr# = 0.50-0.54, and Fe3+/(Fe3++Fe2+) = 0.43-0.52) (Table 1; Figures 3c and 4d to 4f). Type III chromite, with symplectitic texture, is composed of variable proportions of magnetite-rich (Mg# = 0.29-0.33, Cr# = 0.76-0.79, and Fe3+/(Fe3++Fe2+) = 0.62-0.64) and spinel-rich (Mg# = 0.64-0.73, Cr# = 0.41-0.43, and Fe3+/(Fe3++Fe2+) = 0.40-0.54) phases (Table 1; Figures 3d and 4d to 4f). This chromite prior to exsolution have similar Mg# (0.44-0.50) and Cr# (0.54-0.59), but higher Fe3+/(Fe3++ Fe2+) (0.60-0.61) than Type I chromite from disseminated chromitites (Mg# = 0.42-0.52, Cr# = 0.55-0.63, and Fe3+/(Fe3++Fe2+) = 0.43-0.48) (Table 1; Figures 4a to 4c).

Figure 3 Backscattered-electron (BSE) images of the different textural varieties of chromites identified in Los Congos and Los Guanacos chromitite bodies. Type I chromite in massive (a) and semi-massive chromitites (b) with relics of clinopyroxene; Type II chromite from Los Congos (c) and Type III chromite from Los Guanacos (d). Abbreviations: Atg: antigorite, Cb: carbonates, Chr: chromite, Cpx: clinopyroxene, Mag: magnetite, Spl: spinel (Whitney and Evans, 2010).

Figure 4 Compositional variations of chromite grains in the studied chromitites from Los Congos and Los Guanacos in terms of Mg# [Mg/(Mg+Fe2+) atomic ratio] versus Cr# [Cr/(Cr+Al) atomic ratio] (a and d) and Fe3+/(Fe2++Fe3+) ratio (b and e), and Al-Cr-Fe3+ compositions (c and f). Legend provided as inset in the figure. Dashed lines in (a and d) denote the composition of olivine in equilibrium with chromite at a nominal temperature of 1,200 ºC (Dick and Bullen, 1984). Shaded fields in plots are the composition of chromite from Los Congos and Los Guanacos chromitites reported by Escayola et al. (2004) and Proenza et al. (2008).
Table 1 Characterization of the chromitite samples investigated in this study.
Chromite variety | Locality | Sample | Chromitite body | Chromite phase | Chemical variations | |||
---|---|---|---|---|---|---|---|---|
Texture | Silicate matrix | Cr#at | Mg#at | Fe3+ / (Fe3++Fe3+) | ||||
Type I | Los Congos | M-25 | Massive | Atg (10 vol%) | Chromite-rich | 0.47-0.58 | 0.61-0.68 | 0.44-0.54 |
Cpx (10 vol%) | ||||||||
M-17 | Atg (15 vol%) | 0.62-0.72 | 0.52-0.57 | 0.44-0.48 | ||||
Cpx (3 vol%) | ||||||||
Clc (2 vol%) | 0.60-0.73 | 0.48-0.55 | 0.24-0.27 | |||||
Los Guanacos | 3317 | Semi-massive | Atg (35 vol%) | |||||
Cpx, (4 vol%) | 0.55-0.63 | 0.42-0.52 | 0.43-0.48 | |||||
Clc (4 vol%) | ||||||||
2260a | Disseminated | Atg (55 vol%) | ||||||
Cpx (7 vol%) | ||||||||
Clc (5 vol%) | ||||||||
Type II | Los Congos | M-27 | Disseminated | Atg (50-70 vol%) | Spinel-rich | 0.50-0.54 | 0.56-0.63 | 0.43-0.52 |
Cpx, (4 vol%) | Magnetite-rich | 0.85-0.87 | 0.21-0.23 | 0.62-0.63 | ||||
Type III | Los Guanacos | 2226 | Massive | Atg (< 20 vol%) | Spinel-rich | 0.41-0.43 | 0.64-0.73 | 0.40-0.54 |
Magnetite-rich | 0.76-0.79 | 0.29-0.33 | 0.62-0.64 | |||||
Chromite-rich(a) | 0.54-0.59 | 0.44-0.50 | 0.60-0.61 |
Note: Mineral abbreviations after Whitney and Evans (2010).
*a) Composition calculated using the proportion of each phase in exsolved chromites according to Colás et al. (2016).
Type I chromite is found in clinopyroxene-bearing massive, semi-massive and disseminated samples from Los Congos and Los Guanacos massifs, whereas Type II chromite is preserved exclusively in clinopyroxene-bearing disseminated samples from Los Congos, and Type III chromite occurs in clinopyroxene-free massive chromitites from Los Guanacos (Table 1).
The three varieties of chromite are made up by subhedral and less frequently anhedral crystals (from 0.2 to 1 cm across) with rounded shapes, and have a fracture network of variable density (Figure 3); these fractures are often filled with secondary silicates (i.e., antigorite and chlorite), and less frequently with carbonates (Figure 3c). No primary olivine was observed in the chromitites, whereas antigorite and chlorite forms the interstitial matrix between chromite grains (Figure 3). Clinopyroxene are also found intergranular to Type I and Type II chromites (Figures 3a and 3b). Small inclusions of these silicate minerals are scattered within chromite grains.
In situ LA-ICP-MS analyses of Type I chromite in massive chromitites from Los Congos and Los Guanacos show variable albeit similar contents of Ga (10.32-32.70 ppm), Ti (615-1354 ppm), Zn (1282-2853 ppm), and Mn (2890-8215 ppm), higher contents of Sc (1.5-10.07 ppm) and Ni (1012-2386 ppm), and lower contents of Co (287-370 ppm) and V (657-1007 ppm) than those in semi-massive chromitites from Los Guanacos (Ga = 18.96-27.46 ppm, Ti = 746-1449 ppm, Zn = 1974-2508 ppm, Mn = 2540-3172 ppm, Sc = 0.33-1.23 ppm, Ni = 634-851 ppm, Co = 372-397 ppm, V = 1118-1255 ppm) (Figure 5a to 5b). Spinel-rich phase of Type III chromite in massive chromitites from Los Guanacos are enriched in Ga (26.95-30.13 ppm), Co (616-699 ppm) and Zn (5118-6024 ppm), but depleted in Sc (1.03-2.02 ppm), V (598-836 ppm), Ti (302-526 ppm), Mn (3244-4197 ppm) and Ni (1781-2877 ppm) than the magnetite-rich phase (Ga = 9.42-14.88 ppm, Co = 280-407 ppm, Zn = 846-1082 ppm, Sc = 2.19-4.67 ppm, V = 830-1282 ppm, Ti = 876-1378 ppm, Mn = 3097-5083 ppm, Ni = 3700-5812 ppm) (Figure 5c and 5d). In situ analyses of Type II chromite from Los Congos were excluded because the beam size of LA-ICP-MS (~30-50 μm) is larger than the size of the exsolved phases (see Figure 3c).
4.2. Silicate minerals
Antigorite is present in Type I, Type II and Type III chromites (Table 1), but its composition varies in the different ultramafic massifs. Antigorite crystals in the chromitite bodies from Los Congos (n= 9) vary within the following ranges: 42.42-44.66 wt.% SiO2, 36.04-39.33 wt.% MgO, 3.01-3.99 wt.% Al2O3, 1.48-3.19 wt.% FeO, and 0.68-1.39 wt.% Cr2O3. Antigorite from Los Guanacos (n= 12) has relatively higher MgO (37.24-41.12 wt.%) and FeO (1.77-3.30 wt.%), but relatively lower SiO2 (39.71-43.16 wt.%), Al2O3 (up to 0.71 wt.%), and Cr2O3 (0.03-0.26 wt.%) than those from Los Congos (Appendix 2).
Chlorite (n= 8) associated with Type I chromite in chromitites from Los Guanacos (Table 1) have narrowly variable SiO2 (29.01-31.87 wt.%), Al2O3 (17.45-18.36 wt.%), MgO (32.78-34.27 wt.%), and FeO (1.83-2.63 wt.%) (Appendix 2). All the analysed grains contain significant amounts of Cr2O3 (1.94-3.95 wt.%), but their low Fe/(Fe+Mg) ratios (up to 0.04) overlap the compositional field of clinochlore defined by Hey (1954) (Appendix 2).
Clinopyroxene (n= 26) identified in Type I and Type II chromites (Table 1) has a Mg-rich augite composition (Wo28-29 En67-69 Fs3-5) (Appendix 2), but its composition varies in different types of chromite. Clinopyroxene grains which are associated with Type I chromite in chromitites from Los Congos and Los Guanacos (Table 1) show similar Mg# (0.94-0.96), SiO2 (55.29-57.19 wt.%), Al2O3 (2.19-4.06 wt.%) and CaO (12.96-13.89 wt.%), with relatively higher Na2O (0.16-0.36 wt.%) and Cr2O3 (0.55-1.09 wt.%), and lower TiO2 (0.10-0.25 wt.%) and MnO (0.02-0.13 wt.%) than those from Type II chromites in Los Congos (Mg# = 0.93-0.94, SiO2 = 54.53-56.55 wt.%, Al2O3 = 3.50-4.96 wt.%, CaO = 13.22-13.38 wt.%, Na2O = 0.13-0.26 wt.%, Cr2O3 = 0.43-0.72 wt.%, TiO2 = 0.22-0.33 wt.%, and MnO = 0.13-0.15 wt.%) (Appendix 2).
5. Discussion
5.1. Retrograde metamorphic fingerprints in chromitites
Type III chromite prior to exsolution (Mg# = 0.44-0.50) and Type I chromites in disseminated chromitites have lower Mg# (0.42-0.52) with respect to those in massive chromitites (Mg# = 0.61-0.68), expanding between and parallel to the Fo85 and Fo93 contours (Table 1; Figure 4). This trend is similar to that described in non-metamorphosed chromitites from ophiolites elsewhere, where accessory chromites hosted in olivine are enriched in FeO and the olivine interstitial to chromite in chromitite bodies is enriched in MgO (Leblanc and Nicolas, 1992). It could be attributed to the Mg2+↔Fe2+ exchange between chromite and olivine at high temperatures (i.e., 1000-900 °C) during postmagmatic cooling (Irvine, 1967).Thus, a decrease of Mg# at relatively constant Cr# should be expected in the chromite grains from chromitites with lower chromite/silicate ratios. However, this hypothesis is not valid for Type I and Type III chromites prior to exsolution from Los Congos and Los Guanacos because the decrease of Mg# is associated with an increase of Cr# (from 0.47 to 0.73 in Type I chromite and from 0.54 to 0.59 in Type III chromite prior to exsolution) (Table 1; Figure 4a and 4c).
The trend of Cr2O3 and FeO gain coupled with the MgO and Al2O3 loss showed in Type I and Type III chromites prior to exsolution (Table 1; Figure 4a and 4d) is similar to those reported in chromitites from metamorphosed ophiolites elsewhere (e.g., Gervilla et al., 2012; Prabhakar and Bhattacharya 2013; Ahmed and Surour, 2016; González-Jiménez et al., 2016; Kapsiotis et al., 2018; Yu et al., 2019). These chemical variations are usually characteristic of Fe2+-rich porous chromite rims or grains formed by the reaction of magmatic chromite with olivine and/or pyroxene during the retrograde hydrous metamorphism, in presence of SiO2-rich and MgO-poor fluids derived from the host ultramafic rocks (e.g., Bach et al., 2006; Frost et al., 2013; Majumdar et al., 2016; Colás et al., 2017). This hydration process produced the mass loss of primary chromite and the preferential partitioning of MgO and Al2O3 towards chlorite that crystallized inside the pores of Fe2+-rich porous chromite, and also the formation of antigorite in the matrix (e.g., Gervilla et al., 2012; Barra et al., 2014; González-Jiménez et al., 2016; Colás et al., 2019). Interestingly, Type I chromite in disseminated chromitites is significantly more enriched in Co and V and depleted in Ni than chromite in massive chromitites (Figure 5a and 5b). This suggests that a greater element mobility could take place as a result of highly effectiveness in chromite grains with lowest chromite/silicate and high fluid/rock ratios (i.e. semi-massive chromites) (Loferski, 1986; Candia and Gaspar, 1997; Proenza et al. 1999; Merlini et al. 2009; Barra et al. 2014; Colás et al. 2017).

Figure 5 Box chart diagrams illustrating the content of divalent (a and c), trivalent and tetravalent (b and d) minor and trace elements in the different textural varieties of chromite identified in Los Congos and Los Guanacos chromitites. Elements arranged according to Colás et al. (2019).
In contrast, the high contents in Fe2O3 in the Type I and Type III chromites prior to exsolution from Los Congos and Los Guanacos [Fe3+/(Fe3++Fe2+) from 0.24 to 0.54 in Type I, and from 0.60 to 0.61 in Type III chromite prior to exsolution] (Table 1; Figure 4) are usually associated with the modification of Fe2+-rich porous chromite to Fe3+-rich non-porous chromite during the retrograde hydrous metamorphism. This process is a consequence of the exhaustion of olivine and pyroxene from the chromitites and host ultramafic rocks, and the progressive increase of ʄO2 and SiO2 in the fluid (i.e., more oxidizing conditions), which promoted the oxidation of Fe2+ and the subsequent increase of Fe3+ in the system (e.g., Alt and Shanks, 1998; Bach et al., 2004, 2006; Alt et al., 2007). These fluids would have reacted with Fe2+-rich porous chromite through the pre-existing pore network, thus dissolving chlorite and precipitating magnetite in the remaining porosity (e.g., Satsukawa et al., 2015; Colás et al., 2019; Gervilla et al., 2019). The diffusion of Fe3+ and Fe2+ through pores resulted in the development of Fe3+-rich non-porous chromite, obliterating the igneous chemical signature of the pre-existing chromite (e.g., Gervilla et al., 2012; Barra et al., 2014; Colás et al., 2019; Yu et al, 2019). In this context, the lowest Fe3+/(Fe3++ Fe2+) ratios (0.24-0.27) showed by Type I chromite in semi-massive chromitites and the highest Fe3+/(Fe3++ Fe2+) ratios (0.60-0.61) displayed by Type III chromite prior to exsolution (Table 1; Figure 4) suggest an uneven efficiency of the alteration process, which could be significantly affected by the presence of secondary permeability in the chromitites and/or by variable fluid/rock ratios (e.g., Loferski, 1986; Candia and Gaspar, 1997; Colás et al., 2017).
5.2. Prograde metamorphic fingerprints in chromitites
The absence of Fe2+-rich porous chromite and/or Fe3+-rich non-porous chromite rims in Type I chromites and the presence of exsolved textures in Type III chromites (Figure 3) suggest a change in the metamorphic conditions. Type I and Type III chromites prior to exsolution have albeit similar Mg# (from 0.42 to 0.68 in Type I, and from 0.44 to 0.50 in Type III chromite prior to exsolution) and Cr# (from 0.47 to 0.73 in Type I, and from 0.54 to 0.59 in Type III chromite prior to exsolution), but higher (Fe3+/(Fe3++ Fe2+) ratios (from 0.24 to 0.54 in Type I, and from 0.60 to 0.61 in Type III chromite prior to exsolution) than primary chromite reported in Los Guanacos by Proenza et al. (2008) (Cr# = 0.48-0.56, Mg# = 0.61-0.75, and Fe3+/(Fe3++ Fe2+) = 0.30-0.36; Figure 4). According to González-Jiménez et al. (2015), the dehydration of previously hydrated chromitite bodies during the thermal prograde metamorphism may produce the reaction of Fe2+-rich porous and/or Fe3+-rich non-porous chromite with antigorite and chlorite from the pores and/or matrix. This process formed an optically homogeneous chromite with a composition close to igneous chromite -i.e., a chromite with lower Cr2O3 but higher MgO, Al2O3 and Fe2O3 than that formed by the retrograde hydrous metamorphism- in equilibrium with Mg-rich olivine and/or pyroxene (e.g., Padrón-Navarta et al. 2010, 2013).
It is worth noting that Type I chromites from Los Congos and Los Guanacos shows patterns of minor and trace elements with lower Ga and higher Co, Zn and Mn than those chromites from non-metamorphosed ophiolitic chromitites (i.e., chromites form back-arc and oceanic suprasubduction zone (SSZ) mantle; Figure 6a to 6c). This suggests that the distribution of minor and trace elements in Type I chromite was disturbed significantly by the formation of Fe3+-rich non-porous chromite during retrograde hydrous metamorphism, and the subsequent re-equilibrium between those hydrated chromites and silicates during the prograde metamorphism. This is consistent with the fact that the enrichment of Co, Zn and Mn coupled with the depletion of Ga in chromite was interpreted as a result of the formation of chlorite and antigorite by the hydration of chromite during the retrograde metamorphism (Colás et al., 2014). This hypothesis is also supported by (i) the absence of Fe2+-rich porous and/or Fe3+-rich non-porous chromite grains and/or rims in Type I chromites, and (ii) with the fact that these chromite grains have albeit similar distribution of minor and trace elements than Fe3+-rich non-porous chromite in metamorphosed ophiolitic chromitites from the Eastern Rhodopes in Bulgaria (Figure 6d; Colás et al., 2014; González-Jiménez et al., 2015, 2018).
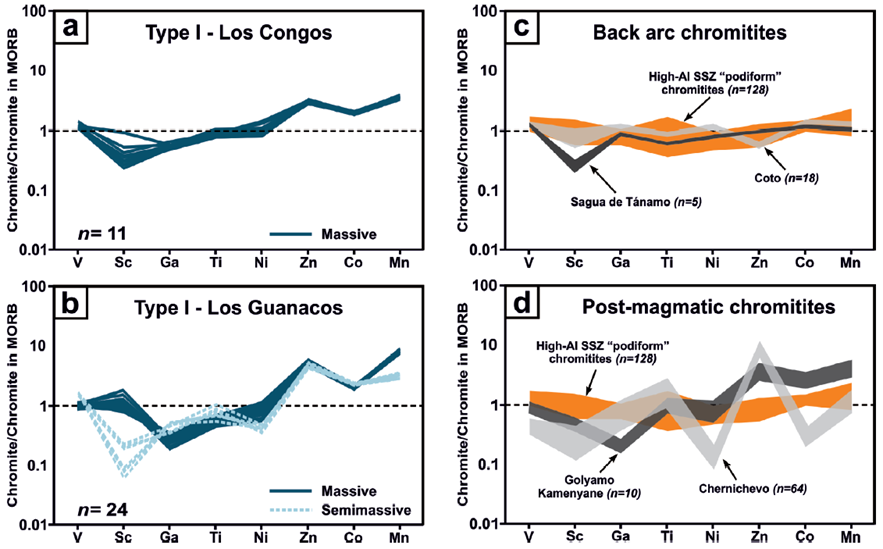
Figure 6 MORB-normalized minor and trace element distribution in Type I chromite grains from Los Congos and Los Guanacos chromitites (a and b) compared with high-Al non-metamorphosed chromitites (c) and with metamorphosed chromitites (d). MORB normalizing values are from Pagé and Barnes (2009). Data sources for high-Al chromites from suprasubduction zone (SSZ) mantle-hosted podiform chromitites: Sartohay, China (Zhou et al., 2014); Islahiya, Doğanşehir (Akmaz et al., 2014) and AntalyaIsparta (Uysal et al., 2016), Turkey; Mercedita (Colás et al., 2014) and Mayarí (Zhou et al., 2014), Cuba. Data source for back arc chromitites is from Coto, Philippines (Yao, 1999), and from Sagua de Tánamo, Cuba (González-Jiménez et al., 2015); and that of from metamorphic chromitites is from Golyamo Kamenyane (Colás et al, 2014) and Chernichevo (González-Jiménez et al. 2018), Bulgaria.
Additionally, Type I chromite in massive chromitites exhibits similar Ga, Ti, Zn, and Mn, higher Sc and Ni, and lower Co and V than that chromite in semi-massive chromitites (Table 1; Figure 5a and 5b). This is consistent with the fact (i) the dehydration of previously hydrated chromitites forms Mg-rich olivine and/or pyroxene with lower Co and higher Ni than Type I chromite (e.g., Zhang et al., 2018; Su et al., 2019), and (ii) the lowest contents of Sc and Fe2O3 [Fe3+/(Fe3++ Fe2+) = 0.24-0.27] (Table 1; Figure 4b and 4c) showed by Type I chromite in semi-massive chromitites is due to the preference of Sc for Fe3+-rich chromites (Wijbrans et al., 2015). Hence, these observations allow us to suggest that the major, minor and trace element composition of Type I and Type III chromites prior to exsolution from Los Congos and Los Guanacos (Figure 4a to 4f) was likely modified by the hydration of primary chromite during the retrograde hydrous metamorphism, and their subsequent dehydration during the prograde metamorphism.
5.3. Temperature conditions of chromite alteration
Recent studies conducted by González-Jiménez et al. (2015, 2016) and Colás et al. (2017) reproduced the phase relations and temperature conditions of the alteration of chromite during the retrograde hydrous metamorphism in the system Cr2O3-MgO-FeO-Al2O3-SiO2-H2O (CrMFASH). However, this thermodynamic approach has limitations to model Fe3+-rich non-porous chromite as Type I, Type II and Type III chromites prior to exsolution from Los Congos and Los Guanacos because the solution model used for Cr-spinel does not include a calibration for the magnetite (Fe3O4) end-member with an inverse spinel structure. Recent refinements of this thermodynamic modelling (González-Jiménez et al., 2018; Colás et al., 2019) now consider that the Fe2O3 content in Fe3+-rich non-porous chromite can be modelled as a magnetite component in the FeO-Fe2O3-MgO-Al2O3-SiO2-H2O (FMASH) system, thus allowing a better constrain the temperature of formation of Fe3+-rich non-porous chromite in chromitites from Los Congos and Los Guanacos.
Such an approach allowed us to construct a pseudosection for Type I chromite, contoured for Cr# and Mg#, in the fluid-saturated FeO-Fe2O3-Cr2O3-MgO-Al2O3-CaO-SiO2-H2O (FCrMACaSH) system (Figure 7). In our computations, we have used an initial assemblage of chromitite bulk composition of semi-massive (57:35:04:04) molar mix of chromite, antigorite, clinopyroxene and chlorite (Table 1). The composition of chromite is the Type I chromite with the lowest Fe3+/(Fe2++Fe3+) ratio [(Mg0.48Fe0.52)(Cr0.72Al0.28Fe0.08)O4], and that of antigorite [(Mg0.48Fe0.52)(Cr0.73Al0.27Fe0.08)O4], clinopyroxene [(Ca0.5Mg1.44Fe0.06)Si2O6] and chlorite [(Mg4.68Fe0.16)Al2Si3(OH)8] is the average composition of those silicates in semi-massive chromitites including Type I chromite (Figure 4a; Table 1; Appendices 1 and 2).
Our modelling shows that the maximum slope of Cr# isopleths in chromite is located between ca. 800 ºC at 20 kbar and 500 ºC at 1 kbar (Figure 7a and 7b), predicting that under water-saturated conditions, primary chromite in semi-massive chromitites is not stable with olivine and pyroxene below 800 ºC. At these conditions, the stable assemblage is made up of chromite with higher Cr# and lower Mg# in equilibrium with chlorite, antigorite, brucite and magnetite (Figure 7a to 7c). The model also predicts that at the P-T conditions for ocean floor metamorphism (4 kbar and 300 °C; Escayola et al., 1996, 2004) estimated for the host ultramafic rocks at Los Congos and Los Guanacos, chromite with Cr# = 1.00 and Mg# = 0.25 in semi-massive chromitite should form (green circle-1 in Figure 7b and 7c). Such modelled values are in accordance with the composition of Fe3+-rich non-porous chromite in metamorphosed chromitites at Tehuitzingo, Loma Baya in southern Mexico (Proenza et al., 2004; González-Jiménez et al., 2015, 2017; Colás et al., 2019; Farré-de-Pablo et al., 2019), Tapo in Peru (Colás et al., 2017), and La Cabaña ultramafic massifs in Chile (Barra et al., 2014; González-Jiménez et al., 2016; Plissart et al., 2019).

Figure 7 P-T pseudosection (a) and isopleths of Cr# (b) and Mg# (c) in chromite and Mg# (d) in olivine calculated for a semi-massive chromitite (57:35:04:04 chromite:antigorite:clinopyroxene:chlorite molar proportions) in the fluid-saturated FCrMACaSH system. Green circles show the metamorphic conditions obtained by Escayola et al. (1996, 2004) in the ultramafic host rocks at Los Congos and Los Guanacos massifs. Orange rectangle show the temperature conditions estimated by Colás et al. (2016) for the exsolution of Type II and Type III chromites and the pressure conditions obtained by Rapela et al. (1998) and Martino et al. (2010) in the associated granulites. Abbreviations: Atg: antigorite, Ath: antophillyte, Chl: chlorite, Chr: chromite, Cpx: clinopyroxene, Hem: hematite, Mag: magnetite, Ol: olivine, Opx: orthopyroxene (Whitney and Evans, 2010).
The occurrence of brucite along with chlorite and antigorite below 500 ºC and 20 kbar further support that ocean floor metamorphism took place under SiO2-poor water-saturated conditions (Colás et al., 2017). Furthermore, the predicted amount of magnetite is <2.58 vol.%, which can be explained as a result of olivine runoff and the oxidation of Fe2+ to Fe3+ in the solutions (e.g., Bach et al. 2004; Alt et al. 2007). This oxidizing fluids circulated through the network of pores in the Fe2+-rich porous chromite, thus dissolving chlorite and diffusing Fe2+ and Fe3+ into the chromite lattice and converts it to Fe3+-rich non-porous chromite (e.g., Gervilla et al., 2012; Barra et al., 2014; Colás et al., 2014, 2017, 2019; González-Jiménez et al., 2016, 2018).
However, Type I chromite in semi-massive chromitites have lower Cr# (0.60-0.73) and higher Mg# (0.48-0.55) and Fe3+/(Fe3++Fe3+) (0.24-0.27) than modelled Fe3+-rich non-porous chromite (Figure 4a to 4c), which suggests a change in the metamorphic conditions (Figure 7a). According to Figure 7a to 7c, an increase in Al2O3, MgO and Fe2O3 could be related to the breakdown of chlorite and antigorite if a prograde metamorphism affects the chromitite bodies. Hence, at the P-T conditions of the metamorphic peak (10 kbar and 800 °C as of Escayola et al., 1996, 2004) (green circle-2 in Figure 7) Fe3+-rich non-porous chromite reacts with chlorite+antigorite+brucite in the matrix. Such reaction of dehydration leads to the formation of homogeneous Fe3+-rich chromite with Cr# = 0.65, Mg# = 0.57 (i.e., Type I chromite), olivine, orthopyroxene and clinopyroxene (Figure 7a to 7c). Clinopyroxene is very rare in non-metamorphosed ophiolitic chromitites, although diopside is found in Al-rich ophiolitic chromitites from the Mayarí-Baracoa Ophiolitic Belt in Cuba (Proenza et al., 1998, 1999; Pujol-Solà et al., 2018), which are spatially associated with gabbro dikes and sills. However, our thermodynamic model also predicts that olivine with Fo93 (Figure 7d) and Mg-rich clinopyroxene (the predicted Mg# is 0.97; Figure 7a) were in equilibrium with a chromite with the composition of Type I chromite in semi-massive chromitites (Figures 4a to 4c and 7).
Significantly, the presence of exsolved textures in Type II and Type III chromites indicate a late cooling from granulite facies metamorphism to lower P-T conditions (Figures 3c, 3d and 7a). Contrary to the petrographic observations, our thermodynamic model cannot predict the exsolution of homogeneous Fe3+-rich non-porous chromite to spinel-rich and magnetite-rich phases in Type II and Type III chromites. However, at the minimum temperature estimated for the exsolution of such chromites (ca. 600 ºC; Colás et al., 2016) and the lowest pressures recorded in the associated granulites (from 4 ± 0.5 to 6.2 ± 1 kbar; Otamendi et al., 1999, 2004, 2005; Rapela et al., 1998; Martino et al., 2010) (orange rectangle-3 in Figure 7), chromite with higher Cr# (0.94-0.98) and lower Mg# (0.36-0.38) than Type I chromite (Figure 7b and 7c) is in equilibrium with olivine, clinopyroxene, chlorite and antophillyte (Figure 7a). The presence of antigorite in the studied chromitites (Figure 3) vs. the predicted occurrence of olivine, clinopyroxene and chlorite by means of thermodynamic modelling (Figure 7), suggests that a final hydration of silicates did not fully progress at amphibolite facies conditions. Further, anthophyllite is not present in the studied chromitite samples (Figure 3), but it was described in ultramafic host rocks to the chromitite bodies at Los Guanacos (Escayola et al., 1996, 2004). Such occurrence can be explained as a consequence of higher rates of reaction between the infiltrating fluids and silicates in chromitites than between these fluids and the host ultramafic rocks. Therefore, these observations suggest (i) Type I, Type II and Type III chromites experienced high-T metamorphism at granulite facies condition after the formation of Fe3+-rich non-porous chromite by the hydrous metamorphic reaction that generated Fe2+-rich porous chromite and magnetite above 650-800 ºC (Figure 7a to 7d; Colás et al., 2019); and (ii) the ultimate mineral assemblage preserved in Type I chromite, as well as the exsolution of Type II and Type III chromites started once the ultramafic body already entered within the P-T field of amphibolite facies (Figure 7).
5.4. Formation of exsolved chromites in chromitites
Colás et al. (2016) suggested that the variety of textures in Los Congos and Los Guanacos chromitites is mainly controlled by the Cr2O3 and Fe2O3 content in chromite (Table 1; Figure 4a to 4f). Under the assumption that Type II and Type III chromites had a similar composition prior to exsolution, such a process could be represented on an ideal phase diagram of chromite (Figure 8). In this diagram, the composition in major elements of Type I chromite and spinel-rich and magnetite-rich phases in Type II and Type III chromites may be represented by an hypothetical solvus on the (Mg0.5Fe2+ 0.5)Cr2O4-MgAl2O4-(Mg0.5Fe2+ 0.5)Fe3+ 2O4 plane, included in the Cr-spinel prism (Figure 8a and 8b) (Tamura and Arai, 2004). As shown in Figure 8b to 8d, the extension of the solvus is more restricted in chromites with high Cr2O3, than in those with low Cr2O3. Therefore, Type I chromite with higher Cr2O3 but lower Fe2O3 contents (Cr# = 0.47-0.73 and Fe3+/(Fe2 ++Fe3+) = 0.24-0.54) than Type II and Type III chromites (Cr# = 0.54-0.59 and Fe3+/(Fe2++Fe3+) = 0.60-0.61) (Table 1; Figure 4a to 4f), should be expected to plot out of the solvus at >600 ºC, which is the blocking temperature calculated by Colás et al. (2016) -i.e., the minimum possible temperature for exsolution (Figure 8b-c). However, Type II and Type III chromites prior to exsolution (i.e., 800 ºC, the metamorphic peak conditions estimated by Escayola et al., 1996, 2004) would be found in the miscibility gap during the cooling from granulite to amphibolite facies, as recorded in the host granulites (Escayola et al., 1996, 2004; Rapela et al., 1998; Otamendi et al., 1999, 2004, 2005; Martino et al., 2010).
In Figure 8d is also shown that Type III chromite prior to exsolution has, in a binary system, an intermediate composition of Fe2O3 (Fe3+/(Fe2++Fe3+) = 0.60-0.61; (Table 1 and Figure 4d to 4f), which lies inside the spinoid at the blocking temperature. Thus, the symplectitic texture in Type III chromite (Figure 3c) could be effectively formed by phase separation of spinel-rich and magnetite-rich phases into the spinoid, as a consequence of a continuous and slow exsolution process through time (e.g., Kingery et al., 1976; Tamura and Arai, 2004). This exsolution process also produced the enrichment in Co, Zn, Sc, and Ga in the spinel-rich phase and the enrichment in Mn, Ni, V and Ti in the magnetite-rich phase in Type III chromite (Figures 5a and 5b). We have assumed that the original composition of Type II chromite was analogue to Type III chromite prior to exsolution (Mg# = 0.44-0.50, Cr# = 0.54-0.59, and Fe3+/(Fe3++Fe2+ = 0.60-0.61; Figure 4a to 4f). This implies that Type II chromite should also plot inside the spinoid at the blocking temperature (Figure 8d). However, the presence of a magnetite-rich phase in Type II chromite as irregular blebs or forming very fine lamellae within the spinel-rich phase (Figure 3d) indicates a discontinuous and relatively fast exsolution process.

Figure 8 Schematic illustration for interpretation of the different textural varieties of chromite identified in Los Congos and Los Guanacos chromitites according to their composition. (a) Cr-spinel prism including the approximate plane for Type I, Type III and Type III chromite compositions. (b) Hypothetical solvus on the Cr-Al-Fe3+ plane calculated by Sack and Ghiorso (1991a). Al3+-Fe3+ planes that cut through the solvus of (b) at different high and low Cr3+ contents equivalent to Type I (c), Type II and Type III (d) chromite compositions (modified from Tamura and Arai, 2004).
The random distribution of magnetite-rich phase blebs along the rims or forming linear strings in Type II chromite grains (Figure 3d) suggests that such blebs are due to a slow cooling, probably at high temperatures (i.e., the metamorphic peak conditions at 800 °C; Escayola et al., 1996, 2004) in which a relatively effective and fast intracrystalline diffusion of cations is favoured. However, the crystallographic control of the magnetite-rich phase lamellae implies that they could form at lower temperatures (i.e., the blocking temperature at ca. 600 ºC; Colás et al., 2016) because the intracrystalline diffusion of cations would be less efficient and slower, which would mean that exsolved phase could only concentrate along crystallographic planes. These two exsolution processes are analogue to those reported in chromites from the Chilas (Pakistan; Jan et al., 1992) and the Eastern Desert (Egypt; Ahmed et al., 2008) magmatic arcs, and from the Iwanai-dake peridotite complex (Japan; Tamura and Arai, 2004, 2005). Therefore, the different textures in Type I, Type II and Type III chromites in chromitite bodies from Los Congos and Los Guanacos are the consequence of: i) gradual variations in the Cr2O3 and Fe2O3 contents during the dehydration of previously hydrated chromitites by prograde metamorphism within the granulite facies, and ii) the cooling rates during the retrograde overprint within the amphibolite facies.
6. Conclusions
The observed textural varieties of chromite in Los Congos and Los Guanacos chromitites in the Eastern Pampean Ranges (north Argentina) were produced during a complex metamorphic evolution. The hydration of magmatic chromite in the course of the retrograde hydrous metamorphism resulted in the formation Fe3+-rich non-porous chromite, and the hydration of olivine and pyroxene to antigorite and chlorite in the matrix. A shift in metamorphic conditions to higher pressures and temperatures (i.e., prograde metamorphism) promoted the dehydration of Fe3+-rich non-porous chromite by its reaction with antigorite and chlorite. This process formed an optically homogeneous chromite with higher Fe2O3, FeO, Co, Zn and Mn but lower Ga than primary chromite by its equilibration with Mg-rich olivine and pyroxene. The later-stage cooling was highlighted by the hydration of Mg-rich silicates in Type I chromite and by the exsolution of Type II and Type III chromites at different cooling rates. A continuous and slow cooling developed symplectitic textures in Type III chromite, thus mobilizing Co, Zn, Sc, and Ga into the spinel-rich phase and Mn, Ni, V and Ti into the magnetite-rich phase. However, a discontinuous and relatively fast cooling produced the exsolution of irregular bleds and fine lamellae in Type II chromites. Thermodynamic calculations showed that the hydration of magmatic chromite to secondary Fe3+-rich non-porous chromite in equilibrium with chlorite, antigorite and brucite occurred below 500 ºC and 20 kbar. Whereas, the dehydration of those minerals forms Fe3+-rich homogeneous chromite and Mg-rich olivine and pyroxene in the matrix above 800 °C and 10 kbar. The subsequent hydration of silicates in Type I chromite and the exsolution of Type II and Type III chromites started at ~600 ºC. Such P-T framework is in agreement with the metamorphic pathway proposed for the evolution of the chromitite-bearing ultramafic rocks at Los Congos and Los Guanacos. This indicates that the prograde metamorphism within the granulite facies conditions exerted large compositional effects on the chromite grains that were previously hydrated during retrograde metamorphism. Therefore, the continent-continent collisional orogenies are the likeliest regional contexts to preserve the geochemical fingerprints of prograde metamorphism in the suprasubduction zone ophiolitic chromitites.