1. Introduction
Calcium carbonate (CaCO3) is one of the most widely used inorganic compounds in several products, such as plastics, papers, sealants and adhesives, cosmetics and toiletries, and construction materials (concrete, plasters, asphalt). CaCO3 is one of the most abundant compounds in nature, being part of several geo-cycles in marine and lake sediments (Lowenstam & Weiner, 1989). Moreover, it is the main constituent of osseous structures, exoskeletons, and protective structures of several species. Mineral CaCO3 can be present in nature as polymorphs, such as calcite, aragonite, vaterite, and ikaite, or as amorphous CaCO3 (ACC), with the stability decreasing in this order under environmental conditions (Cartwright, Checa, Gale, Gebauer, & Sainz‐Díaz, 2012). With respect to the CaCO3 crystal formation process, in the classical theory of crystal growth, CaCO3 formation has been depicted as dependent on supersaturation conditions and temperature, which in turn, promote reactions to create different CaCO3 polymorphs. Although the classical theory of biomineralization is still developing, the key factors are: 1) ion species under supersaturating conditions; 2) high hydroxyl concentration; and 3) availability of nucleation sites (Dhami, Reddy, & Mukherjee, 2013), which could be supplied by living organisms. Bacterial CaCO3 mineralization is a phenomenon that occurs in sediments, caves, and even monuments and buildings (Aguilera, Zapata, & Morales, 2015; Ciferri, Tiano, & Mastromei, 2000; Rusznyák et al., 2012). The ability of bacteria to nucleate calcium ions and form minerals is explained by the function and nature of exopolymeric substances (EPS) and biofilm geometry (Dupraz, Braissant, Decho, Norman, & Visscher, 2009). In this context, bacterial biofilm geometry and composition has been related to microorganismal metabolism (Decho, 2010), since it forms a microenvironment to concentrate nutrients present in the medium. Some studies have concluded that the nature and composition of biofilms have an impact on morphology, bioaccumulation, and CaCO3 formation mechanism, which determines crystal geometry (Andrei et al., 2017; Li et al., 2015; Rodriguez-Navarro, Jroundi, Schiro, Ruiz-Agudo, & González-Muñoz, 2012). More recently, a plethora of research groups have isolated different bacteria possessing the capacity to precipitate CaCO3 (Arias, Cisternas, Miranda, & Rivas 2019; Dhami Navdeep, Mukherjee, & Watkin, 2018; Kim et al., 2016; Seifan, Samani, & Berenjian, 2016), including diverse species of the Bacillus genus. Bacillus licheniformis, Lysinibacillus sphaericus, and Bacillus sphaericus have been shown to produce CaCO3 crystals with different morphologies (Seifan et al., 2016). Moreover, CaCO3 with different polymorphisms have been obtained from Pseudomonas putida INQCS 113, Lysinibacillus sphaericus INQCS 414, and Bacillus subtilis INQCS 328 (Shirakawa, Cincotto, Atencio, Gaylarde, & John, 2011), and the precipitation of vaterite by B. pumilus has also been reported (Daskalakis et al., 2015). Several strains of B. subtilis, a naturally occurring bacteria found in soil, have been isolated via its capacity to bioprecipitate CaCO3 (Han et al., 2019; Montoya, Márquez, López, & Cuervo, 2005; Schwantes-Cezario et al., 2017) and employed as a model to describe the molecular mechanism involved in CaCO3 bio-precipitation (Perito et al., 2000). Furthermore, Marvasi et al. proposed the involvement of the B. subtilis etfA gene in the bio-precipitation of CaCO3 through its participation in the synthesis of long-chain fatty acids. EtfA mutants were shown to produce a lower amount of dipicolinic acid, which modified the phospholipid composition of the membrane and rendered bacteria unable to precipitate CaCO3. Therefore, membrane composition could play an important role in providing nucleation sites (Marvasi, Casillas-Santiago, Henríquez, & Casillas-Martinez, 2017). On the other hand, another study found that fragmented cells can induce mineral precipitation of CaCO3 (Perito et al., 2014).
The development of novel cement-based materials for building is one of the most trending applications of the biomineralization process. The durability of cement-based materials, such as concrete and reinforced concrete, can be increased by implementing microbial calcite, porous filler, coating systems, and self-healing systems (Kalhori & Bagherpour, 2017; Nguyen, Ghorbel, Fares, & Cousture, 2019; Park, Kim, & Ghim, 2012; Perito et al., 2014; Schwantes-Cezario et al., 2019; Schwantes-Cezario et al., 2020; Tayebani & Mostofinejad, 2019a). Specifically, with respect to the control of reinforced concrete corrosion, some electrochemical techniques, including the application of potentiostatic pulses, have proven effective (Bastidas, González, Feliu, Cobo, & Miranda, 2007); and as a consequence, electrochemical methods have been proposed for the mitigation of corrosion in reinforced concrete (Bastidas, Cobo, Otero, & González, 2008). On the other hand, novel construction materials have been developed by biocementation and bioconsolidation of limestone bricks, sand, and other cement-based materials. The biocementation capacity of Bacillus species has been exploited to develop a biogrouting system in wellbore soil and rocks, which has been applied to reduce leaking (Cunningham et al., 2014; Phillips et al., 2018; Wu, Chu, Wu, & Hong, 2019). Moreover, it has been found that B. subtilis can form a CaCO3 coating on the surface of materials exposed to corroding environments, increasing their durability (Nosouhian, Mostofinejad, & Hasheminejad, 2015). However, in many studies, urea degradation is the main pathway for the induction of calcium bioprecipitation, which leads to ammonia liberation and the production of bad odors. On the other hand, the use of CaCO3 as a calcium source in this biogenic approach could have negative effects in the application of reinforced concrete due to the presence of chloride ions, which may promote corrosion processes. Furthermore, although some investigations have proposed the application of hydroxyapatite and other phosphates as corrosion inhibitors in the presence of chloride ions for reinforced concrete (Bastidas et al., 2010), the biogenic approach to creating durability of cement-based materials remains an interesting challenge. Accordingly, the objective of the present study was to characterize the formation of crystals by Bacillus subtilis in a semi-solid non-ureolytic system, avoiding the formation of chloride ions and focusing on the potential applicability to construction biomaterials and the development of a novel protective system with self-healing ability.
2. Experimental - Materials and Methods
2.1. Calcium carbonate biogenic production
Experiments was performed using Bacillus subtilis ATCC 23857 strain. Medium composition was nutrient broth (0.5% peptone and 0.3% beef extract) for propagation and inoculums. Biogenic production was performed in nutrient agar supplemented with 0.025 M calcium acetate. Petri dishes was inoculated by triplicated, incubated at 37°C for 3 days.
2.2. Calcium crystal recuperation
Agar petri dishes were washed with 11 mL with ultrapure water at room temperature. A soft scraping was performance in agar surface in order to obtain crystals. Water was recuperated, and by decantation crystals was recovered. Two ultrapure water washed was performed until supernatant was transparent. Solvent excess was evaporated during 48 hours at 37°C.
2.3. Crystal morphology analysis
Obtained crystals were treated with silver/palladium sputtering and then observed by scanning electronic microscopy (SEM) in a JEOL JSM-7000F Field Emission Scanning Electron Microscope equipped with an energy-dispersive X-ray spectroscope (EDS). EDS mapping was performed to confirm and verify crystal composition. Working distance was of 11 mm and the excitation voltage was 20 KV.
2.4. Detection of Calcium Carbonate allotropic forms
Crystals were characterized by X ray diffraction (XRD) by monocrystal technique, Fourier Transform Infrared Spectroscopy (FTIR) was applied to quantify the different variations of calcium carbonate allotropic forms. XRD measurements were performed from 0 to 40 2Θ degrees scanning with molybdenum radiation. The step size was 0.22 Theta degree and the Kα value was 0.71073 Å. FTIR measurements were performed by Bruker alpha platinum - ATR spectrophotometer.
3. Results and discussion
3.1. Analysis of crystal morphology and surface composition
Cultured crystals were recovered and dried after a period of 3 days. Crystal size and morphological structure were observed by microscopy (Figure 1). The crystals exhibited a heterogeneous size varying from 50 to 170 µm (114 ± 40 µm) and a rounded morphology with a defined border and pyramidal center. Moreover, a high birefringence was observed, suggesting that the crystals were composed of CaCO3.
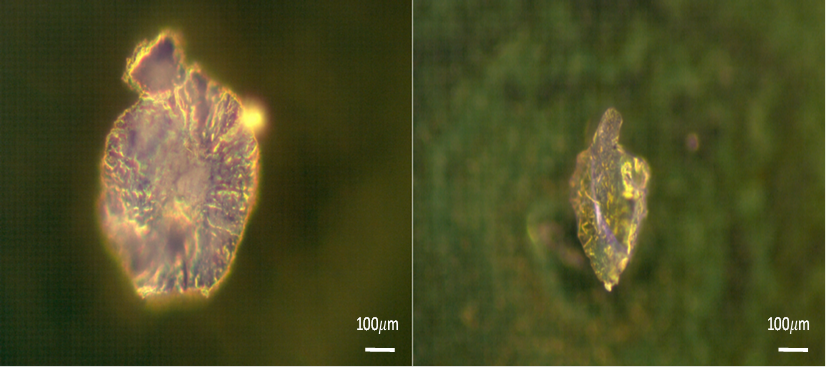
Figure 1 Reflected polarized light microscopy images of crystals obtained by biomineralization of Bacillus subtilis culture after 3 days.
The crystal growth pattern showing central and radial expansion corresponded to the Bacillus subtilis colonial morphology, and the biofilm can be considered a scaffold for crystal growth. Bacillus subtilis is a Gram-positive bacteria, and its capacity to produce several exopolymeric substances is well known. An important structure of B. subtilis is the cell wall, which consists of peptidoglycans and teichoic acid, providing an electronegative charge due to the presence of functional groups such as COO- and PO4 -3 (Schultze-Lam, Fortin, Davis, & Beveridge, 1996; Voběrková, Hermanová, Hrubanová, & Krzyžánek, 2016). During biofilm formation, the bacterial capsule exhibits permeability to metallic ions. Polar amino acid residues in the inner part of the cell provide hydrophobic properties (Schultze-Lam et al., 1996). There are sufficient anionic groups still exposed and strategically located in the cell wall, such that exopolymeric substances can interact with dissolved metals, which may be responsible for the presence of nitrogen in crystals. In the same manner, surfactin can interact with calcium ions, reducing the surface tension and therefore decreasing colony size (Mhatre et al., 2017).
Top-view SEM images were obtained to characterize the morphology of biogenic crystals over a culture period of 3 days (Figure 2). In the inferior section, mineral nucleation can be appreciated, which likely occurred by precipitation that eventually formed smooth surfaces. In the upper part, mineralized cells and surrounding mineral nucleation can be observed; a similar effect has previously observed (Rodriguez-Navarro et al., 2012). The crystal predominant phase was found to be laminar calcite, which is in accordance with earlier reports (Andrei et al., 2017; Rodriguez-Navarro, Jroundi, Schiro, Ruiz-Agudo, & González-Muñoz, 2012). As stated in some investigations, biomineralization begins near the cell surface since bacterial metabolic processes increase the pH of the surrounding microenvironment. Subsequently, a passive precipitation process occurs, in which calcium ions (Ca2+) interact with exopolymer substances and cellular structures present in the biofilm, using exposed radicals as nucleation sites (Decho, 2010). Crystal formation occurs around cells due to the interaction of Ca2+ with the cell wall (Marvasi, Visscher, Perito, Mastromei, & Casillas-Martínez, 2010), the effect of which can be appreciated in Figure 2. Biofilm interaction promotes cell fossilization (Figure 2).
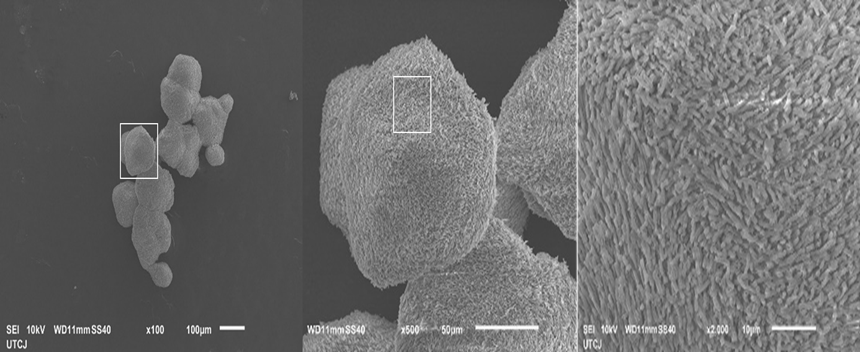
Figure 2 Top-view SEM images of crystals obtained by biomineralization of Bacillus subtilis culture.
The elemental composition of the biogenic crystals was determined by energy-dispersive X-ray spectroscopy (EDS) to confirm that the crystals were indeed composed of CaCO3. The results show the presence of carbon, calcium, and oxygen elements, as illustrated in Figure 3.
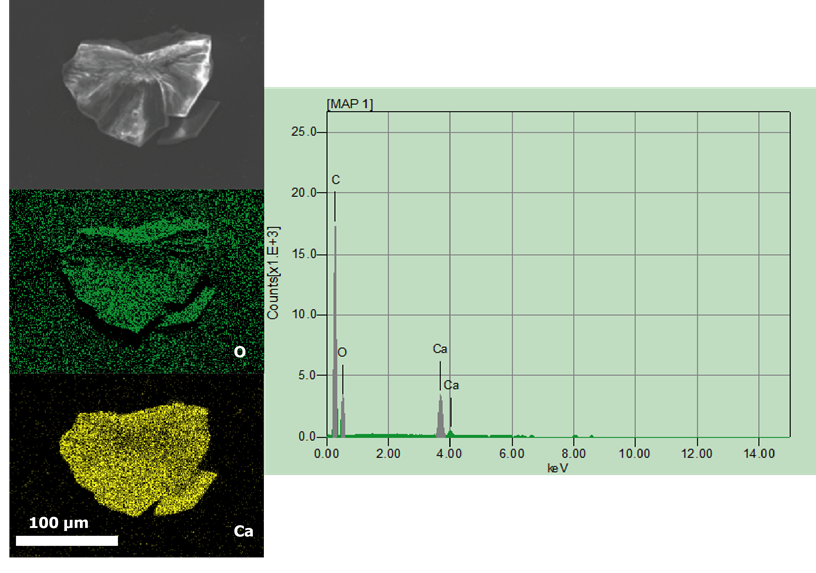
Figure 3 Top views and EDS mapping elemental analysis of crystals obtained by biomineralization of Bacillus subtilis culture after 3 days of exposure.
Regarding the crystalline phase, it has been proposed that spatial distribution is influenced by biofilm characteristics and physicochemical properties of the microenvironment, in addition to the nature of exopolymeric substances produced by bacteria. Moreover, according to previous studies, it is postulated that both the capsule and surfactin molecules are responsible for the promotion of crystal precipitation (Li et al., 2015). Finally, as Ca2+ accumulates and interacts with surfactin, it inhibits Bacillus subtilis biofilm expansion by reducing the viscosity (Mhatre et al., 2017), which is considered another factor that directly impacts crystal morphology.
3.2. Crystallinity and crystal phases
Crystallinity was quantitated and characterized by the surface analysis techniques, X-ray diffraction (XRD) and Fourier-transform infrared (FTIR) spectroscopy. XRD allows determination of mineralogical composition (Badillo-Camacho et al., 2016), and in combination with FTIR spectroscopy, allows characterization of crystallinity. The XRD results were analyzed using the Panalitycal X'Pert Highscore Plus software, and quantitation of crystalline and non-crystalline phases was performed by Rietvield's refinement using atomic coordinates (Bish & Howard, 1988; Chung, 1974; Wang & Becker, 2009). The XRD spectrum was compared with that of calcite, vaterite, and aragonite standards; however, a peak pairing correlation of the sample with standard patterns was unsuccessful (Figure 4).
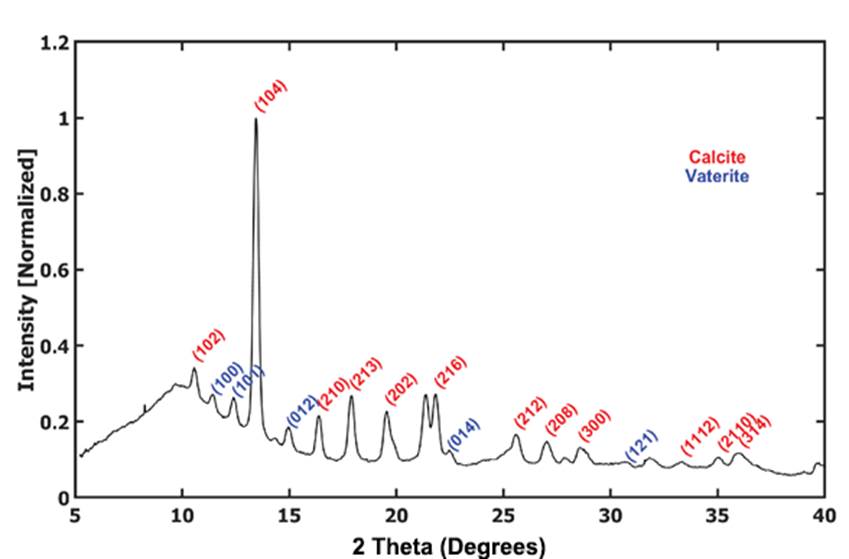
Figure 4 X-ray diffraction patterns of calcite CaCO3 and vaterite CaCO3 polymorphs obtained by biomineralization of Bacillus subtilis culture after 3 days of exposure. biomineralization of Bacillus.
The crystalline and non-crystalline phase distribution of the crystals observed in Figure 4 shows that calcite was the predominant phase at 82.2%, with the remaining phase being vaterite at 13.8%, the former of which constitutes the most metastable phase under environmental conditions (Lowenstam & Weiner, 1989). Crystallinity was calculated by an area detector using the EVA software, which demonstrated a value of 81.1%. Moreover, amorphous material was present in the crystal and is ascribable to its biogenic origin, at which ion nucleation begins with an amorphous arrangement because the crystal is hydrated. As the crystal dehydrates, it is transformed to a more crystalline phase until it reaches a calcite polymorph (Gong et al., 2012; Schultze-Lam et al., 1996). The predominant crystalline phase is calcite and to a lesser extent aragonite, with vaterite being the most unstable phase, although it tends to change to a much more stable phase like calcite. Vaterite can be stabilized by biological molecules (Rodriguez-Navarro et al., 2012); nevertheless, the mechanism and factors influencing the polymorphism formation during bacterial bio-precipitation remain unclear. The highest intensity reflection was plane 104 for calcite and plane 213 for vaterite. The mean crystal size was calculated using the Scherrer equation and determined to be 12.36 nm and 12.45 nm for calcite and vaterite, respectively. Small crystalline sites could be related to the nucleation sites, at which CaCO3 could be formed near the cell wall, leading to the production of micritic growth, a very common process in the formation of microbialites (Perri & Spadafora 2011).
Biogenic CaCO3 crystal formation evidenced amorphous CaCO3 (ACC) as the precursor, from which CaCO3 crystalline polymorphisms were precipitated (Cartwright et al., 2012). The calculated amorphous phase percentage of the crystals was 18.9%. The stability of amorphous material may be related to the interaction of precipitates with bacterial biofilms, allowing metastability toward environmental conditions (Aizenberg, Lambert, Weiner, & Addadi, 2002). The results concerning stabilization of the amorphous phase are attributable to the presence of the biofilm, since the cell wall and exopolymeric substances can act as a matrix in a similar way to the proteins present in bivalve shells and other organisms that use organic matrixes for phase stabilization (Cartwright et al., 2012).
To corroborate bacterial crystal phases, the absorption spectrum was measured by FTIR spectroscopy as presented in Figure 5. Three maximum absorption bands were observed, identifying calcite at 873 cm-1, aragonite at 712 cm-1, and an amorphous phase at 1406 cm-1. Lower intensity bands were also found as calcite at 1089 cm-1, aragonite at 854 cm-1, an amorphous phase at 1130 cm-1, associated water at 630 cm-1, and vaterite at 745 cm-1. However, the vaterite band was at extremely low intensity as shown in several references (Montoya et al., 2005; Ni & Ratner, 2014).
4. Applications in novel cementitious materials
New demonstrated and potential applications related to calcium carbonate precipitation by biomineralization process of Bacillus species have been proposed in approaches like biocementation for development of new construction materials and self-healing of civil structures. Biocementation have been used successfully to reduce the concrete porosity, protect from corrosive environments, repair microcrack and improve mechanical properties of the material (Joshi, Goyal, Mukherjee, & Reddy, 2019; Karimi & Mostofinejad, 2020). Several Bacillus species are able to promote the precipitation of CaCO3, when the fixation of calcium by biomolecules occurs in the bacterial biofilm, by converting environmental CO2 to HCO3- via enzymatic activity, which promotes an alkaline environment through the degradation of nitrogen sources such as proteins, urea, and NO2 (Dupraz et al., 2009). In this respect, it is worthwhile mentioning that biogenic CaCO3 precipitation only occurs in an alkaline environment (pH > 8) (Joshi, Goyal, Mukherjee, & Reddy, 2017). As a consequence, this biogenic CaCO3 does not decrease the pH and therefore will not corrode steel when considering its application in reinforced concrete. Schematic representation of bacterial mechanism is shown in Figure 6 to illustrate pH increase as part of biopreciptation process.
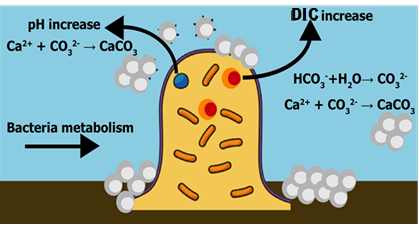
Figure 6 Schematic representation of bacterial metabolism during CaCO3 precipitation: pH and dissolved inorganic carbon (DIC) increase.
4.1. Development of novel self-healing materials
Investigations using the above-mentioned approaches have been conducted with a view to developing a special type of concrete referred to as ‘bacterial concrete,’ in which bacteria are induced to mortars and concretes to heal micro-cracks as they appear.
The development of self-healing materials by microbial-induced precipitation has been examined in several studies using bacteria of the Bacillus genus, showing an increase in the durability and mechanical properties of the concrete and a decrease in chloride permeation (Dessai, Panandikar, Ramarajan, & Pillai, 2019; Hussein, Abedali, & Ahmead, 2019; Nugroho et al., 2019; Tayebani & Mostofinejad, 2019b). Regardless, as mentioned previously, several studies have used ureolytic bacteria and CaCl2; however, degradation of urea produces ammonia as a bad odor and CaCl2 can be harmful to reinforced concrete structures due to the addition of Cl-1, which is responsible for corrosion.
The development of novel cement-based materials for the building industry is one of the most trending applications of the biomineralization process. Repair systems for concrete and reinforced concrete failures, cement binders, porous fillers, coating systems, and self-healing systems in cementitious materials are novel approaches that will avoid failure of structures due to fractures (Andalib et al., 2016; Jonkers, Thijssen, Muyzer, Copuroglu, & Schlangen, 2010). Demonstration of this implementation on a pilot scale has been successful, decreasing water permeability by 47% 2 months after application (Wiktor & Jonkers, 2015). However, there are certain factors that adversely reduce the potential application of bacteria as repair systems; for example, bacterial survival under the environmental and physicochemical conditions of concrete.
It is noteworthy that when urea is used in conjunction with a calcium source in a self-healing approach, production of ammonia is the result, which may increase the risk of steel corrosion following application to reinforced concrete (Abdullah, Abdullah, & Tompang, 2019). In addition, because of the fact that concrete is exposed to ingression of chloride or sulphate ions, which can also reduce the self-healing ability of bacterial concrete, these parameters need to be explored prior to practical implementation. To enhance the survival rate of bacteria inoculated in cementitious material, researchers have proposed the incorporation of other ceramic materials such as inoculated aggregates and encapsulated nutrients and bacteria (Chen, Qian, & Huang, 2016). Therefore, for self-healing applications, the durability of bacteria in the presence of detrimental substances should be investigated a priori. Investigations of some novel self-healing concrete using Bacillus gene are presented in Table 1.
Table 1 Novel investigations of self-healing concrete / biocement mortar. Using the Bacillus gene.
Bacillus gene | Approach | Improvement | Ref. |
---|---|---|---|
Bacillus subtilis | Self-healing concrete | Self-healing of cracks of (<0.5 mm) | (Khaliq & Ehsan, 2016) |
Bacillus pasteurii | Self-healing concrete | Use of lightweight aggregates as bacterial carriers. Crack repairmen effect. | (Chen, Peng, Tang, 2019) |
Bacillus cereus | Biocement production rich-urea | Use of urease for maximum production of biocement | (Anitha, Prakash, Seshagiri, Rao, & Vanavil, 2018) |
Bacillus subtilis | Biocement mortar | Compressive strength of cement mortar | (Abdulkareem, Fadeelat, Zaimi, Rahman, & Jay, 2019) |
4.2. Development of novel cementitious materials using biocementation process
Some investigations have addressed the possibility to engineer the mechanical properties of this biogenic carbonates in cement-based materials (Dhami et al., 2013). Development of new construction materials by biocementation and bioconsolidation of limestone bricks, sand and other cementitious materials have gained attention by using the biocementation process of Bacillus species (Joshi et al., 2019; Zamarreno, Inkpen, & May, 2009). The biocementation ability process of Bacillus species has been used for installing a biogrouting system in wellbore soil and rocks, and it has been implemented in systems to reduce leaking of lixiviation (Wu et al., 2019). Examples of the application of Bacillus gene in biocmentation process for development of novel cementitious materials are illustrated in Table 1.
Another important type of cement-based concrete, self-compacted concrete (SCC) is produced with the same ingredients of normal concrete and these cementitious materials are also adopted to replace the cement content in SCC in order to use waste materials from industries, agricultural products. However, to further enhance the performance of SCC, and to reduce the formation of micro-cracks, investigations have been directed towards the inclusion of specific bacterial cell concentration, like Bacillus species. SCC is considered to have many advantages in comparison with conventional concrete like improved construction quality, faster construction activity, reduced cost, among others.
The importance of understanding the genetic factors related to urease and carbonic anhydrase and their associated regulatory mechanisms has been realized with respect to the use of this approach in the development of bioconcrete (Castro-Alonso et al., 2019). Future work focused on these aspects will incorporate the development of novel bacterial strains by genetic engineering to improve their survival and activity under the harsh conditions of concrete and reinforced concrete.
4.3. Monument biorestoration
In 2002, a mechanism using bacteria to conserve monuments through the technique of bio-mineralization was proposed (Perito & Mastromei, 2003). Microbial CaCO3 precipitation was also investigated for the reinforcement of monumental stones, yielding successful results (Barabesi, Salvianti, Mastromei, & Perito, 2003). Exploiting the ability of bacteria to precipitate CaCO3 has also been proposed for the repair and restoration of monuments and historic heritage buildings due to the compatibility of CaCO3 with marble and limestone (Perito et al., 2014; Rodriguez-Navarro et al., 2012). It is important to mention that the carbonation process through biomineralization has been referred to as microbial-induced calcium carbonate precipitation (MICCP) for the conservation of carbonate stone monuments and historic building materials (Nazel, 2016).
Conservative intervention in monument restoration by crystal precipitation using Bacillus subtilis has been analyzed in small bioclastic limestone samples, and the genetic mechanisms that control calcite precipitation have been investigated. Data suggest the presence of several genes involved in crystal formation (Perito et al., 2000). Since the biodeterioration process involves every type of cultural heritage item, including monuments, stonework, frescoes, and easel paintings, the potential use of microorganisms as restorative agents in biological control has recently been realized as a ‘sustainability approach.’ In this regard, new investigations including Bacillus-based bioactive compounds have been proposed (Soffritti et al., 2019). This biorestoration approach includes biocleaning and bioconsolidation, which applies the bio-calcifying capabilities of Bacillus species. The most recent studies focusing on the applicability of Bacillus-based compounds in biorestoration are summarized in Table 2.
Table 2 Novel biorestoration of monuments with the Bacillus gene.
Bacillus gene | Approach | Implementation | Ref. |
---|---|---|---|
Bacillus spp | Decontaminating against biodeteriogenic fungi | Immersion | (Silva, Rosado, Teixeira, Candeias, & Caldeira 2015) |
Bacillus subtilis | Bioconsolidation of historical limestone | Spraying | (Micallef, Vella, Sinagra, & Zammit, 2016) |
Bacillus spp | Decontaminating activity against biodeteriogenic bacteria | In vitro test | (Casselli et al., 2018) |
Bacillus spp | Biocleaning from historical Bricks | Spraying | (Casselli et al., 2019) |
5. Conclusions
In this investigation the biomineralization of calcium carbonate by Bacillus subtilis yielded two allotropic forms of crystals, both amorphous and crystalline, during only 3-day period of incubation in solid media. Crystals were identified as calcite and vaterite phases. Morphology indicated that bacteria influenced mineral nucleation around their cell wall. Crystal phase distribution has been reported for similar biological mineralization induced by another Bacillus species. These results open a broad opportunity for implementation of Bacillus-based bioactive compounds with biotechnological perspectives with a sustainability approach of biogenic restoration and reinforcement of monuments. Biogenic carbonates derived from Bacillus gene would be emerging as sustainable alternative cement-based materials and have been proposed for application in the protection of materials by self-healing ability such as the anticorrosion process in case of reinforced concrete. While many approaches for biodeposition of calcium carbonate using Bacillus-based bioactive compounds have been focused on published sources, there are considerable possibilities for new research.