1. Introduction
Fiber reinforced polymers (FRP) are widely used in different sectors, such as aeronautics, construction, wind energy, and the automotive industry, due to their high specific properties, low weight, and ease to manufacture parts of different sizes and forms (Bohmann et al., 2018; Keck & Fulland, 2019; Ramón-Valencia et al., 2015). The most commonly used options, as a complement to FRP, are glass fibers (GFRP) and carbon fibers (CFRP). On one hand, fiberglass is the most common due to low cost and lower fragility, meanwhile carbon fiber has a higher quality in terms of mechanical properties (Nor et al., 2018). The use of this type of materials is considered as a viable alternative to substitute steels and aluminum alloys (Riccio et al., 2018). For example, CFPR application in a car bodywork can save up to 10 wt% compared to aluminum, provides lightness, reduces CO2 emissions and fuel consumption (Liang et al., 2019).
However, synthetic fibers have huge environmental problems: a high manufacture energy-consumption and difficult waste management at the end of its life cycle (Dehghan et al., 2017). Because of this, the use of natural plant fibers as FRP reinforcement has aroused great interest in the scientific community (Liu et al., 2019; Mora-Espinosa & Ramón-Valencia, 2018). In fact, the number of performed studies has increased exponentially year after year, since these types of fibers offer great benefits, such as: sustainable production(recyclable, renewable or compostable), competitive advantages (low price, processability, and diversity), ecological advantages (low toxicity, low tributary emissions and low-energy consumption), and abundance in waste disposal options (Maradei-García et al., 2019; Noryani et al., 2019). Within the most studied group of natural fibers are included: sisal, ramie, cotton, flax, jute, bamboo, coir, hemp, pineapple leaf, and rice husk (Li et al., 2019). Fibers such as jute, flax, hemp and coconut, are a good reinforcement in polymer matrices, and they are used in automotive applications, construction, and packaging (Ramesh et al., 2017).
For example, Cadu et al. (2019) studied the influence of hygrothermal aging under humidification and drying cycles, on the properties of a flax-based composite. Authors recorded an approximate 10% decrease in final Young's modulus and 14% in final tensile strength, after one year of exposure and 52 aging cycles. This showed promising results for this type of biologicall-based materials (Cadu et al., 2019).
The study of Pereira et al. (2017) is another example, where continuous jute fibers and unidirectionally aligned were used as reinforcement of an epoxy resin matrix. The obtained biocomposite was tested under the Charpy test, in order to determine the impact properties. Results showed an improvement in the energy-absorption capacity, as the volume fraction of jute fiber increased. It should be noted that the highest energy-absorption value was 214 J/m (with 30% by volume of jute fiber), which is among the best obtained results so far by lignocellulosic fiber composite (Pereira et al., 2017).
On the other hand, jute is one of the most used natural fibers as reinforcement in green composites, showing significant advantages over other fibers, such as low cost and a maximum production volume around 2.3 to 2.85 million tons per year. In addition, its fine texture, and its heat and flame resistant provide a wide range of industrial applications in sectors as: textiles, construction and the automotive industry (Singh et al., 2018). In general, its tensile strength has an average value of 583 MPa, surpassing fibers such as ramie (500 MPa) (Arpitha & Yogesha, 2017), bamboo (290 MPa), banana (355 MPa), cotton (400 MPa), henequen (430 MPa), werregue (249 MPa), and caña flecha (168 MPa) (Mora-Espinosa & Ramón-Valencia, 2018).
It is important to highlight that the automotive industry is one of the main industrial sectors, where the social, environmental and governmental needs or responsibilities are often taken into consideration. The use of vegetable fibers as reinforcement materials, in the field of automotive engineering, has advantages such as: recyclability, good thermal and acoustic insulation properties, biodegradability, low cost, availability, energy recovery, less tool wear in machining operations, and less skin and respiratory irritation (Ramesh et al., 2017).
Consequently, this research was carried out with jute-polyester biocomposite viability demonstration purposes, as a substitute for the traditional material used in the interior opening handle from a car door. For this purpose, samples of the biocomposite were manufactured using the vacuum assisted resin transfer moulding (VARTM) technique, which matrix is a polyester resin and the reinforcement is a biaxial fabric (90°) made with jute fiber. Then, tensile and flexural tests were carried out on standardized test pieces under ASTM specifications, in order to mechanically characterize the jute-polyester biocomposite. Subsequently, a finite element-based static analysis was performed in the ANSYS software, to determine the mechanical behavior of an interior opening handle from a car door. A model sensitivity study was run to determine the influence of the mesh type and identify its convergence. Later, static analysis results were obtained: critical zone, maximum operating stress and safety factors. Finally, a scale model of the piece made with jute-polyester biocomposite was manufactured.
2. Materials and methods
2.1. Biocomposite raw material
Unsaturated polyester orthophthalic thermosetting resin was used as matrix, marketed under the reference P-2002 by the company Industria de Resinas SAS. The manufacturer recommends the use of methyl ethyl ketone peroxide (MEKPO) as a catalyst.
Jute fiber layers, bi-directionally woven at 90° with a density of 1100 g/m2, were used as reinforcement (Figure 1). The jute fiber layers were dried at a temperature of 95 °C during 20 minutes, before the biocomposite manufacturing process.
2.2. Biocomposite manufacturing process
Vacuum Assisted Resin Transfer Moulding (VARTM) was used as a manufacturing process, as shown in the scheme in Figure 2. Acrylic plates were used as mould for the manufacture of the laminates, using interlayers of jute fibers. Subsequently, the resin/catalyst mixture was made in a ratio of 100:1 wt parts. Once the system was installed, negative pressure of 0.8 bar was applied with a vacuum pump. Finally, the obtained laminates were placed in a controlled environment at 25 °C temperature for 7 days.
2.3. Destructive test
Tensile test was performed under ASTM D3039/D3039M specifications (ASTM D3039/ D3039M, 2017), with 5 specimens of 250x25x2.5 mm, gage length of 100 mm, and the rate of crosshead motion at 1 mm/min. To comply with the thickness requirements, the biocomposite was laminated with 3 layers of jute fiber.
Three-point bending was carried out under ASTM D790 specifications (ASTM D790-17, 2017), with 5 specimens of 135×13×3.5 mm, support span of 80 mm, and the rate of crosshead motion at 1 mm/min. In this case, 4 layers of jute fiber were applied to obtain the required thickness. Mechanical tests were carried out on a universal SHIMADZU UH-600KN machine, making use of uniaxial tensile tests accessories, as well as in bending experiments.
Thermosetting resin P-2002 without reinforcement mechanical properties were taken from the study carried out by Velasco et al. (2020), to determine the influence of jute reinforcement (Velasco-Parra et al., 2020).
2.4. Finite element-based static analysis of an interior opening handle from a car door
Traditional static analysis has served as starting point for the knowledge of mechanical requirements of any element. However, the geometry complexity of some parts limits the development of simple analytical models, and it makes it difficult to acquire information related to work stresses. In addition, the main purpose of the industrial sector is to reduce the time and cost of product manufacturing (Rahman et al., 2009). Therefore, the finite element method (FEM) was used, with ANSYS simulation software. This in order to predict the loads and critical areas of an interior opening handle from a car door, and validate the use of the jute -polyester biocomposite as a substitute material
A finite element-based static analysis flowchart is shown in Figure 3. In the Computer-Aided Design phase, a detailed three-dimensional model of an interior opening handle from a car door was developed using SolidWorks software. For Finite Element Model phase, ANSYS software was used.
In this pre-processing stage, the loads, supports and mesh were included.
The operating conditions were set (Figure 4a), where: A and B are cylindrical faces representing the piece pivot axis; C and D are fixed supports representing the restriction of position of the car door opening mechanism; and E is a force of 20 N representing the applied load by the user hand. Likewise, ten node tetrahedral elements (TET10) with mean size of 0.3 mm were used for the solid mesh (Figure 4b). For the mesh global length selection, Finite Element Analysis results were taken into account, such as: total nodes, total elements, element quality, and equivalent stresses. These values were analyzed in the Model Sensitivity Study, where the described mesh global length was obtained. Finally, Material Mechanical Properties were included in the Static Analysis Results (Figure 3), in order to obtain the minimum Safety Factor in the mechanical piece critical zones and validate the use of the biocomposite in the automotive application.
3. Results
3.1. Tensile test
Tensile mechanical properties (Table 1) and stress-strain curves (Figure 5) of polyester P-2002 with and without woven jute fibers have been analyzed.
Table 1 Tensile properties of materials.
Properties | Polyester P-2002 |
Jute-polyester biocomposite |
---|---|---|
Young’s modulus (GPa) | 0.61±0.12 | 0.89±0.37 |
Tensile strength (MPa) | 31.11±3.97 | 40.50±8.70 |
Tensile strain (%) | 5.10±1.60 | 5.67±1.61 |
Results of the mentioned analysis show that tensile strength of biocomposite containing woven jute fibers with polyester P-2002 matrix is found to be 40.50 MPa. Tensile strength of virgin polyester is 31.51 MPa, lower value compared with the biocomposite. Further, it has been found out that a significant increase exists in the Young’s modulus with the addition of natural fibers to the polyester matrix. In P-2002 with addition of woven jute fiber, the Young’s modulus increases 46% up to 0.61 GPa value.
On the other hand, elastic-linear behavior was observed with a maximum value of stress in the break point of both materials. Figure 6 corroborates the biocomposite brittle fracture. It is important to note that thermoset matrix nature is fragile and, therefore, biocomposite material adopts the same behavior. However, in relation to strain, the increase is favorable when natural fibers are incorporated, with a variation of 11% respecting virgin polyester. This modification can be interpreted as a contribution of the natural fibers to the biocomposite, due to the elastic properties that these natural species present. This phenomenon is reflected in the orientation of the fibers after the material fracture, during the tensile test (Figure 6).
Figure 7 shows a comparison of the tensile strength of the developed jute-polyester biocomposite, with other materials reinforced with jute fiber (Gopinath et al., 2014; Ray et al., 2020; Sowmya & Ramesh, 2018). The proposed material outperformed, by far, tensile strength results of the short jute fiber reinforced composite and the polypropylene-matrix jute woven fiber material. This shows thermosetting matrix and woven fiber configuration has the best properties respecting obtained tensile stress. Also, it was evidenced that jute-polyester is not competitive compared to the hybrid material with hemp fibers, but it has an outstanding tensile strength.
3.2. Flexural test
Flexural mechanical properties (Table 2) and stress-strain curves (Figure 8) of polyester P-2002 with and without woven jute fibers have been analyzed.
Table 2 Flexural properties of materials.
Properties | Polyester P-2002 |
Jute-polyester biocomposite |
---|---|---|
Flexural modulus (GPa) | 1.30±0.25 | 1.54±0.21 |
Flexural strength (MPa) | 45.23±5.34 | 107.42±34.26 |
Flexural strain (%) | 5.89±1.72 | 6.43±1.86 |
Flexural modulus and flexural strength values of virgin polyester increased by 18% and 137%, respectively, with the addition of jute woven fiber layers. Similarly, biocomposite bending strain increased by 9% compared to polyester. As a result, the jute-polyester biocomposite obtained a flexural strength (or modulus of rupture) of 107.42 MPa and a flexural modulus of 1.54 GPa.
On the other hand, stress-strain curves demonstrated once again elastic-linear behavior of the studied materials, with thermosetting matrix brittle fracture (Figure 9). In this case, fibers performed better under flexural stresses and, as can be seen in Figure 9, most of them did not break after the destructive test. This confirmed obtained results in the tensile tests, where jute woven fibers improved the biocomposite elastic behavior. Furthermore, it was observed jute-polyester biocomposite was better able to withstand flexural stresses than tensile stresses. This factor was favorable, due to the combination of stresses to which the automotive part under study is subjected.
Figure 10 shows the flexural strength comparison of the developed jute-polyester biocomposite, with other reinforced materials with jute fiber (Gopinath et al., 2014; Ray et al., 2020; Sowmya & Ramesh, 2018). Referenced investigations also concluded flexural properties exceed tensile properties when jute-fiber reinforcement is used. Again, it is shown that thermosetting matrix with jute woven fibers configuration, exceeds expectations of short jute fibers or thermoplastic matrix composites. Furthermore, in the case of flexural stresses, the jute-polyester biocomposite can even compete in resistance with the hemp-jute hybrid/polyester composite.
3.3. Model sensitivity study
Model sensitivity study made feedback from Finite Element Model, taking into account to obtain the best piece discretization. This depended on calculated results precision and their convergence. For this purpose, the variables were monitored, depending on the mesh global length assigned to the model in Figure 4. In addition, ANSYS automatic tetrahedral mesh approach was used, using two types of elements: four nodes (TET4) and ten nodes (TET10). According to Rahman et al. (2009), Tetrahedral elements are recommended, because they produce high-quality meshes, for limits discretization of most solid models imported from CAD systems (Rahman et al., 2009).
In Figures 11 and 12, it can be appreciated that quality and total elements is inversely proportional to the mesh global length. In addition, there is a similarity in the obtained results, with maximum quality values of 0.84 and more than 1.2 million elements. This shows that node configuration in TET4 and TET10 elements does not influence mesh adaptability in the CAD geometry.
On the other hand, Figure 13 shows the increase in nodes number present in the geometry, as the mesh global length decreases. The TET10 mesh obtained greater number of nodes along the different mesh global length, compared to the TET4 mesh. Here, the difference of nodes with the global mesh size of 0.3 mm was more than 1.5 million.
This notable increase in nodes number becomes important when obtained results are analyzed in Figure 14, which shows critical value monitoring of the equivalent (von Mises) stress, for both cases (TET4 and TET10). It is evident that TET10 mesh predicted higher stress values than TET4 mesh, in all mesh global length. The mentioned above justifies that nodes number used has a significant influence on stress prediction. Therefore, TET10 mesh was used to perform general static analysis of the interior opening handle from a car door.
3.4.1. Identification of mesh convergence
Figure 15 shows the solutions for two types of critical stresses, at different TET10 mesh global length: equivalent (von Mises) stress and maximum principal stress. According to obtained values, it is evident that when maximum principal stress is used, obtained results in the simulation are more demanding, compared to those calculated in the equivalent (von Mises) stress. In addition to this, model convergence point was determined, with global mesh sizes less than 0.3 mm. Therefore, maximum principal stress based on the TET10 mesh with an element size of 0.3 mm (1268500 elements and 1839525 nodes) was chosen. This as the parameters of FE model for results acquisition of the mechanical performance of the interior opening handle from a car door.
3.4.2. Simulation and solution
Figure 16 shows maximum principal stress distribution in the interior opening handle from a car door.
Likewise, piece critical zone location (Max) is shown, which stress value reached
19,528 MPa (
It should be noted that, if the material meets critical zone requirements, it has a superior performance in the rest of the piece. This is mainly due to safety factor; it is a design approach that takes into account modelled physical system uncertainties and provides error margin between material strength and critical conditions present in the application.
In Table 3, safety factor values are observed, respecting tensile and flexural properties of the materials. Flexural safety factor values were higher than traction values, as a result of higher composite performance against this type of stress. On the other hand, polyester resin without reinforcement shows acceptable values, jute-polyester biocomposite presents even higher performance with an increase of 30% in tensile safety factor, and 137% in flexural safety factor.
Table 3 Minimum safety factor located in the critical zone.
Properties | Polyester P-2002 |
Jute-polyester biocomposite |
---|---|---|
Tensile safety factor | 1.59 | 2.07 |
Flexural safety factor | 2.32 | 5.50 |
It should be noted that the main drawback of using natural fiber composites in industrial applications is their moisture absorption characteristic (Arputhabalan et al., 2019). Many researchers have shown that temperature and water absorption percentage cause a decrease in mechanical properties of this type of material (Sujon et al., 2020; Srinivasan et al., 2020). Models have even been developed to describe damage caused to the stiffness and resistance of the composite material reinforced with natural fiber swollen by water absorption in service conditions (Tian & Zhong, 2019).
However, obtained results with respect to the safety factor reflect, in the worst case (tensile safety factor of 2.07), material properties can reduce up to 40%, and even so, it is suitable for part operating conditions. Therefore, error margin is located within acceptable design parameters for this type of application (Chang, 2015). In addition, biocomposite heterogeneous nature is irrelevant, and obtained results computationally validate the use of this new material as a viable substitute for the manufacture of an interior opening handle from a car door.
3.5. Manufacturing of interior opening handle from a car door
In Figure 17a, the mould used to manufacture the interior opening handle from a car door is observed, taking the real car part as a model. Mould Part A has the resin inlet and part B has the vacuum outlet and cavity for four jute fiber layers. Layers number was selected according to results shown in Table 3, where jute-polyester biocomposite flexural properties reached safety factor maximum value. Part manufacture process was Vacuum Assisted Resin Transfer Moulding (VARTM), and it obtained the interior opening handle from a car door (Figure 17b). Piece final mass was 20 grams, only 1 gram above original opening handle obtained in polypropylene.
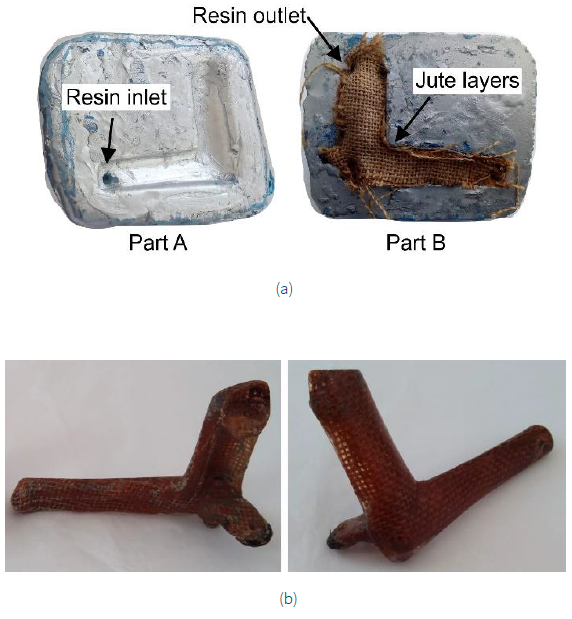
Figure 17 Manufacture of the interior opening handle from a car door: (a) mould assembly, and (b) jute-polyester biocomposite part.
Figure 18a shows a comparison between the assembly of the original polypropylene part and the one made with jute-polyester biocomposite. Figure 18b shows the inside of the door, where the handle was fitted to the opening mechanism. Once installed, correct operation of the interior opening handle from a car door made with jute-polyester biocomposite was verified. However, it is still necessary to carry out experimental tests on the final part to verify its life cycle.
4. Conclusions
In this research paper, polyester resin was combined with layers of bidirectional (90°) reinforcement of jute fiber. Tensile and flexural tests were carried out under ASTM specifications, to mechanically characterize jute-polyester biocomposite, and compare it with polyester resin without reinforcement. Finally, finite element-based static analysis was performed in ANSYS, to computationally validate the jute-polyester biocomposite use, in the interior opening handle design from a car door.
According to tensile and flexural test stress-strain curves, it was found that jute-polyester biocomposite has an elastic-linear behavior with a maximum strength greater than its thermoset matrix. This means that jute fiber reinforcement provides a significant increase in mechanical properties, and jute-polyester biocomposite failure is fragile due to the polyester-resin nature. Furthermore, flexural properties shown higher values than tensile properties, in both cases. These results were verified with other investigations, demonstrating a good competitive level against other types of jute fiber reinforced composites.
Finite element-based static analysis had great importance to predict the mechanical behavior of the interior opening handle for a car door. Model sensitivity study was very useful to determine mesh parameters, obtaining most demanding stress values in the critical zone. Similarly, obtained safety factors in finite element-based static analysis evidenced that the proposed material is capable of supporting the operating loads.
Obtained results computationally validate the use of jute-polyester biocomposite, as a substitute for the manufacture of an interior opening handle from a car door. Consequently, a piece scale model was made and assembled on the car door. Although the life cycle of the final part remains to be analyzed through experimental tests, jute-polyester biocomposite undoubtedly has a promising future and may be viable for parts manufacture for the automotive sector.