Introduction
Climate change presents considerable challenges for forest managers and ecologists in terms of practicing effective management activities for either commercial or conservation purposes. Ongoing climate change has already generated heat waves, droughts and precipitation events of unprecedented intensity and frequency (Hansen, Sato, & Ruedy, 2012).
As a consequence of climate change, models predict that suitable climatic habitats for most of the current North American biomes will either shift to more northerly latitudes, climb to higher elevations, expand (xeric biomes, such as dry deciduous forest), shrink (several temperate and high altitude biomes, such as alpine forest) or disappear (rare and humid biomes, such as cloud forest) (Aitken, Yeaman, Holliday, Wang, & Curtis-McLane, 2008; Rehfeldt, Crookston, Sáenz-Romero, & Campbell, 2012; Rehfeldt et al., 2014).
In this paper, we present and discuss indications that the shift in geographic location of suitable climatic habitat for forest tree species because of rapid climatic change will be likely to cause important stresses on natural tree populations. Due to the physical limits of natural migration (the speed at which processes such as seed dispersal, establishment, competition and sexual maturation can take place), tree populations will be challenged to keep pace with the moving target that their suitable climatic habitat will become. We address the following questions: In order to accommodate climate change, what forest management options do we have? What would be the consequences of inaction, i.e., continuing as if climate change does not exist? We aim to provide foresters with a framework of basic knowledge regarding the magnitude and speed of climate change and its current and potential effects on forest resources, as well as suggesting some options for active management aimed at maintaining healthy tree ecosystems in the future.
Predictions of climatic change
It is expected that climate change will increase annual average temperatures and modify precipitation patterns. In many cases, precipitation will decrease (generally inland, while it may increase along some coastlines). In general, the expected temperature increases will be of greater magnitude towards the poles (as high as 6.9 °C by year 2090) compared to near the equator (as low as 1.8 °C), relative to a normalized period (1961-1990 or 1951-1990) (Hansen et al., 2012; World Meteorological Organization [WMO], 2013).
Differences in climate change predictions among the various greenhouse gas emission scenarios are greater for the distant future (end of the century), depending on the model assumptions related to the growth rates of the world economy and human population and to advances in technology (Intergovernmental Panel on Climate Change [IPCC], 2000). However, there is less doubt regarding the accuracy of temperature increases predicted for the near future (around 2030; Sáenz-Romero et al., 2010). Actions must be taken to ensure that the forests harvested today are re-planted with genetic resources that are adapted for the temperatures predicted for the period centered around 2030 or at most 2060, in order to avoid the excessive forfeiture of growth that will occur if these trees are planted on a site where suitable climate will not occur until too far ahead into the future (Ukrainetz, O’Neill, & Jaquish, 2011).
Climatic change that has already occurred
For various reasons, it is difficult to say how much the climate has already changed: (a) Consensus is required on what constitutes the period of reference and it can be difficult to obtain complete meteorological records over large geographic areas for that particular period, b) Intrinsic climatic variability exists from year to year, (c) Climate change has produced an increase in within-year variability and (d) Climate change is not uniform; it presents complex regional patterns (Hansen et al., 2012).However, even considering all of the above factors, general patterns do emerge. Global mean annual land (oceans excluded) temperature was 0.8 °C warmer in the decade 2000-2010 than during the period of 1961-1990. This land temperature increase was greater in the northern (0.9 °C) than in the southern (0.5 °C) hemisphere (WMO, 2013).
The incidence of extreme temperatures has increased dramatically: in the last 30 years, extreme high temperatures occurred over ten times more often than was the case before 1950. This means that heat waves (three standard deviations above the average of maximum temperatures for the 1951-1990 base period), occurred over 10 % of the Earth´s land surface over the period 1981-2010, while such extreme heat events occurred on average over only 1 % of the land surface between 1951 and 1990 (Hansen et al., 2012).
Of great concern, however, is that such a trend will continue because of the continuing accumulation of CO2 in the atmosphere. In preindustrial times, the level of atmospheric CO2 was 280 ppm, but it averaged 362 ppm in the decade 1991-2000, 380 ppm in the decade 2001-2010 and 389 ppm in the year 2010 (WMO, 2013) and 397 ppm in 2014 (Hansen & Sato, 2015). There has certainly been no indication of any decrease in the rate of atmospheric CO2 accumulation.
Basic concepts of adaptive genetic differentiation among forest tree populations
Forest tree species and populations have evolved to adapt to the environment in which they grow (Rehfeldt, 1988). This evolution occurred at both species and population levels. Typically, populations of widely distributed forest tree species differentiate genetically in order to adapt to the climate (Rehfeldt et al., 2002), soil conditions and factors of disturbance (e. g., outbreaks of fire and pests) that prevail where they grow (Alfaro et al., 2014). Genetic differentiation means that populations of the same species can differ from each other in one or more traits that allow them to survive, grow, compete and reproduce in a given environment. Examples of such adaptive traits are the timing and rate of growth, resistance to frost damage or to drought stress, seed set and dispersal (distance and timing).
The result of this microevolution of populations in montane regions, for example, is that within the same forest species, populations from low altitudes can grow more and for a longer period of time during the frost-free season, but are more susceptible to frost damage during the cold days that can occur in late spring or early autumn. In contrast, populations from high altitudes grow less and over a shorter period of time and are much more resistant to frost damage (Rehfeldt, 1988).
Decoupling of populations from the climate for which they are adapted
Climate change is expected to decouple forest tree populations from the climate to which they are adapted. In other words, the climate for which they have evolved will occur in a different place or may even disappear entirely (Rehfeldt et al., 2012). This will create physiological stresses because of the increased temperatures combined, in many cases, with reduced precipitation. It is likely that the trees will be much more susceptible to attack by endemic insects and diseases, the populations of which have always been present, but the trees will be weakened and thus less able to successfully cope with the attack. Although phenotypic plasticity (plant ability to respond to environmental change) may mitigate the impacts of decoupling, the capacity for response may be exceeded and the stress induced by environmental factors could prove to be too strong for the plants (Mátyás et al., 2010).
Evidence from packrat middens (from fossilized plants on waste food rat sites) clearly indicates that, at least initially with climate change, plant species extirpation far exceeds immigration, leading to an impoverished flora (Betancourt, 1990). In fact, there are numerous documented examples of sudden forest decay attributable to climatic changes that have already taken place, mostly at the low altitudinal limits or at the southern limit of species distributions (in the northern hemisphere). For example, a massive forest decline killed 12,000 km2 of Pinus edulis Engelm. (pinyon pine) in southwestern USA during the drought period of 2000-2003 (Breshears et al., 2005), a sudden decline of Populus tremuloides Michx. (aspen) was observed in the Rocky Mountains, USA (Rehfeldt, Ferguson, & Crookston, 2009), likewise for Cedrus atlantica (Endl) G. Manetti ex Carriére in the Moyen Atlas mountain range, Morocco (Mátyás, 2010) (Figure 1) and for Fagus sylvatica L. (beech) in South-west Hungary (Mátyás et al., 2010). In Catalonia, in northeastern Spain, declining species are already being replaced by more drought-tolerant species, as can be seen in the example of F. sylvatica being replaced by Quercus ilex L. (Peñuelas, Oyaga, Boada, & Jump, 2007).
Can tree population distributions keep pace with climate change?
There is a common belief among the general public that nature allows species to evolve and populations to adapt to environmental changes (such as the glaciations) and that therefore this should occur once again in response to climatic change (Hansen, 2009). This belief is mostly wrong, at least for tree populations. The problem is the speed of the current, human-induced climate change (Hansen, 2009). One way to visualize the problem is to measure the speed in the past at which tree populations have tracked the climate for which they are adapted, e.g., at the end of a period of glaciation, and then compare that with the speed at which these populations are moving at present (Aitken, Yeaman, Holliday, Wang, & Curtis-McLane, 2008; Savolainen, Pyhajarvi, & Knurr, 2007). For example, Fagus grandifolia Ehrh. (American beech) and Acer rubrum L. (red maple) moved north by less than 100 m per year following the last glaciation, but tracking current climate change would require a much faster rate of movement (McLachlan, Clark, & Manos, 2005). Biomes would need to move more than 1 km per year to track the suitable habitats predicted for the next 100 years, even without considering water barriers and human development (Malcolm, Markham, Neilson, & Garaci, 2002). Some populations of Pinus sylvestris L. would need to move between 700 and 1500 km (from central-southern Russia towards Siberia) in order to track the climates predicted for the year 2090 (Tchevakova, Rehfeldt, & Parfenova, 2005). This represents a movement of approximately 12.2 km per year. Based on these examples, in order to successfully track these climates, geographic shifts of tree populations will have to be 10 to 100 times faster than they have been in the past or are at present.
In mountain regions, tracking predicted habitats will require upward altitudinal movement. In fact, some tree populations are already moving upwards by natural means, but at a lower rate than that required to keep pace with climate change. Several woody plant and grass species of the forest in some mountains in France have moved upwards by an average of 2.9 m per year over the last 30 years (although averaging woody and grass species); however, tree species move much more slowly than grass species and their average shift has been lower than 2.9 m per year (Lenoir, Gégout, Marquet, de Ruffray, & Brisse, 2008). Considering that the mean annual temperature has increased by 0.9 to 1.0 °C in the alpine regions of France since the early 1980s (Lenoir et al., 2008), and assuming a lapse rate (rate of temperature change for a given change in altitude) of 0.5 °C per 100 m of altitudinal difference, an upward shift of approximately 180 m in 30 years, or the equivalent of 6 m in altitude per year, would be required.
The upper altitudinal limit of F. sylvatica in the Catalonian Pyrenees, Spain, has moved an average of 43.5 m in a period of approximately 80 years, which is equivalent to a shift of about 0.5 m per year. Mean annual temperature has increased by 1.5 °C since 1950 (Peñuelas et al., 2007). Considering the same temperature lapse rate, an upward shift of populations of 6 m per year would be required to account for the shift in climate since 1950. To conclude from the above examples, the natural upward shift in altitude would need to take place at a rate that is between two and 12 times faster than is currently the case. There are also other examples where the vegetation presents no migration and this even occurs in the Arctic, where the largest increases in temperature are observed. This might be due to the natural isolation that acts to limit the expansion of new species in this region (Prach et al., 2010).
Assisted migration, assisted colonization or assisted relocation
The inescapable conclusion is that, for a large number of tree species, human intervention will be needed to match (realign) the populations to the environment to which they are adapted. This human assisted realignment has been called assisted migration, assisted colonization, assisted relocation or facilitated migration (Aitken, et al., 2008; Hewitt et al., 2011; Pedlar et al., 2012; Rehfeldt et al., 2002; Tchebakova et al., 2005).
In general, seed and seedling movement must be upwards and towards the respective poles of each hemisphere. This can be achieved by management practices such as collecting seeds from a given natural population, using these to produce seedlings in a nursery and planting them in a location at which a suitable climate is predicted at a given future time period (not at the original provenance). This shift of seed sources is needed to account for recent past climate change as well as that expected during the lifetime of the planted tree. In other words, healthy trees well adapted to these conditions must be present in order to produce seeds and seedlings that can survive and mature in these future conditions. Indeed, these may well need to be moved again in the future if climate change continues and the natural migration rate is still too small. This proposed management strategy breaks one fundamental concept of classic restoration ecology: that local seed sources are normally best. It seems that this is no longer true for many tree populations.
Planning assisted migration
Planning of assisted migration requires knowledge of the relationship between contemporary populations and their climate, and only then to predict where and when such climates will occur in the future and make decisions regarding when to move what population and to where. For example, based on estimates of the current and future (year 2030) suitable climate for Mexican pine populations distributed in Michoacán state (central-western Mexico), it was concluded that in order to match the climate predicted for the year 2030, an upward shift of 300 to 400 m would be required to compensate for a predicted temperature increase of 1.5 °C (Sáenz-Romero et al., 2010). This means that, if the process begins now, an upward movement of 16 m per year will be required, considering the long generational period of pine species and the age of sexual maturity, however, it might be more effective to move the populations by means of a reforestation program as soon as possible. For example: collect seeds in early 2015, produce seedlings in 2015-2016 in a nursery and plant these in the field during the rainy season of 2016, in a place where suitable climate is predicted for the 2030s. By the target year of 2030, these individuals would be around 15 years old and, assuming the program is successful, the planted trees would then be producing seeds. These seeds could in turn begin to naturally establish a new population adapted to its new environment and thereby contribute to shifting the allele frequencies of surrounding local populations, or they could be collected and used in a further round of assisted migration. In the latter case, a new target climate for a future date of, for example, 2060 (hopefully predictions made in 2030 for 2060 will have more certainty than those made currently for 2060) might once again have to be identified.
Suitable climate habitat models can be built using more complex techniques, such as modeling the presence-absence of species of interest based on selection of relevant climatic variables. For example, contemporary and future (2060) suitable climatic habitats were modeled for Pinus chiapensis (Martínez) Andresen, a rare Mexican pine, and the maps produced can be used to plan assisted migration (Sáenz-Romero et al., 2010; Figure 2). In other words, the development of species or population climate niche models under the contemporary climate could be used to project the future location of the climate niche; seed collection and seedling production could then be guided using the modeled current niche to plant in the future niche.

Figure 2 Predicted suitable climate habitat for Pinus chiapensis, at the eastern slopes of Sierra Madre Oriental, central-east Mexico; probability: yellow 50 to 80 %, red > 80 %; filled circle: actual population. (A) Current climate, (B) year 2060. Arrow in panel B indicates the assisted migration required from a current seed source to match a suitable climate predicted for 2060. Note from the relief that the suggested movement implies towards a higher altitude; Citlaltepetl (also known as Pico de Orizaba) is the highest volcano in Mexico. Modified after Sáenz-Romero et al. (2010).
Modified seed zones for accommodating climatic change
Population movement for reforestation purposes has been a component of forest management for centuries. The initial goal of this action was simply to find the best source of seed; there were no thoughts or concerns about climate change or the need for assisted migration at that time. Seed sources were moved extensively until mortality and productivity losses in plantations, compared to adjacent naturally regenerated stands, became evident in many places (Isaac, 1949). A system for certification of the stand origin of forest tree seeds was established in Washington and Oregon states by the mid-1960s and seed zone maps were published in 1966. These maps were widely used and have served the purpose of ensuring the availability of adapted planting stock for reforestation and restoration. There is ongoing research and discussion directed towards the modification of such seed zones, aiming to collect seeds in a given seed zone but deploying the seedlings in a planting zone that is predicted to present a climate in the future that is similar to that of the zone in which the seed was collected.
The Ministry of Forests, Lands and Natural Resource Operations of British Columbia has amended its seed transfer guidelines to compensate for recent and future climatic change (O´Neill et al., 2008). In most class A and B (seed orchard and wild stand, respectively) seed zones, the upper elevation limit of seed transfer has been increased by 200 m to account for projected climate warming. Historically, seed transfer guidelines in British Columbia generally attempted to match the contemporary climates of the seed source and the planting sites. Under these new guidelines, current seed source climates will be similar to the projected future climate of planting sites.
In mountainous regions, altitudinal seed zones have been projected for contemporary and future climates. For example, it was suggested that seeds from the Mexican tree-line pine Pinus hartwegii Lindl., should be collected in the altitudinal band of 3,150 to 3,350 masl, but that the seedlings produced in nurseries from such seed should be planted at sites from 3,550 to 3,750 masl (an average of 400 m higher in altitude) in order to compensate for the climatic change predicted for the decade centered on the year 2030 (Loya-Rebollar et al., 2013) (Figure 3).

Figure 3 Suggested upward assisted migration of Pinus hartwegii populations at Pico de Tancítaro National Park, Michoacán, western Mexico, to match the climate predicted for the year 2030. Note that seeds collected in Zone 3 (high altitude) might have nowhere to go (because of the summit height in some mountains). These seedling would have to be translocated to other higher mountains. Zone 1 (low altitude) would need to be planted with a different species, such as Pinus montezumae Lamb., which is naturally distributed immediately below P. hartwegii in altitude (Based on Loya-Rebollar et al., 2013).
Selection of species for assisted migration
Selection of species to be moved by assisted migration is complicated in countries of extensive biodiversity, such as Mexico or Colombia. Which species should be chosen for relocation? It is clear that not all species can be moved. One option would be to give priority to those that are already on the brink of extinction; such is the case in three rare, endemic and endangered Mexican spruces: Picea chihuahuana Martínez, Picea mexicana Martínez and Picea martinezii Patterson, which present relictual, fragmented, inbred populations in northern Mexico. The contemporary and future suitable climate distributions of these species were delimited. Counterintuitively in this case, assisted migration needs to be towards more southern locations, since the highest mountains in Mexico are to the south of the current population locations (Ledig, Rehfeldt, Sáenz-Romero, & Flores-López, 2010) and also because Mexico is predicted to become more arid towards the North (Sáenz-Romero et al., 2010). This is a good example of why it is useful to define migration distance using climate variables and not simply in terms of geographic distances.
It has also been proposed that the species of greatest economic interest and/or those that constitute keystone species that provide the structure of a plant community (generally tree species) should have priority for assisted migration (Hewitt et al., 2011). It can thus be expected that when populations of such keystone species are established, they will provide suitable habitat for several other species, including fauna.
Assisted migration at northern latitudes
The climatic diversity of Ontario province, Canada, supports an ecological gradient that includes (from north to south): taiga and cold wetlands in the far north, boreal forest, northern deciduous forest, and eastern deciduous forest in the south. More than 80 tree species are native to one or more of these ecological regions, all of which reach either or both of the northern or southern limits of their contemporary natural range. Given the northern location of Ontario, the most obvious concern is that the early and sustained climate change expected for high latitudes will cause contraction (extirpation) of the southern boreal forest and that the speed of climate change will exceed the capacity of the northern boreal and deciduous forest species to colonize the emerging suitable habitat. Associated management issues include the determination of methods by which to delay the decline and extirpation of the southern boreal forest, but a subtler concern is that locally adapted populations of native tree species will be unable to adapt in situ. While the concerns are clear, the requisite management response is uncertain. This uncertainty centers on two factors; a) the speed and geographic heterogeneity of climate change, and b) the individualistic response of species to incremental changes in climate.
A recent case study of the eastern white pine (Pinus strobus L.) has documented an approach to strategic planning for assisted migration (Joyce & Rehfeldt, 2013). The case study described and mapped climatypes (sensu Rehfeldt & Jaquish, 2010) nested within the P. strobus species climatic niche model. Three General Circulation Climatic Models were then used to project the future geographic distributions of these climatypes. Four seed zones (yellow, green, blue and red - see Figure 4a) were employed to match climatypes to both contemporary and future redistributed suitable habitat. Unexpectedly, the projections for the distribution of suitable habitat for 2060 (Figure 4b) illustrate that the persistence of the most northerly ‘red’ climatype is problematic, while the central (blue and green) and southern (yellow) climatypes are projected to be most suitable to the north and west of their current distribution.
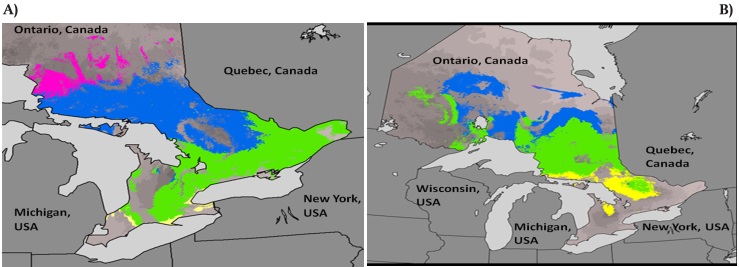
Figure 4 Four seed zones or climatypes (from south to north: yellow, green, blue and red), derived from subdividing the predicted suitable climatic habitat of Pinus strobus (eastern white pine) on the basis of field and common garden provenance tests of the performance of 112 seed sources from east of Lake Superior, Ontario, Canada, for: (A) current climate (average 1961-1990) and, (B) climate projected for the decade centered on the year 2060, using the Geophysical Fluid Dynamic General Circulation Model (GFD-GCM) and the A2 emission scenario (elevated greenhouse-effect gas emission scenario). Modified from Joyce and Rehfeldt (2013).
Matching climatypes to habitat that will be suitable by the mid-century involves modifying existing seed transfer guidelines to collect seeds in current seed zones and subsequently deploy the reforestation stock to the geographic area predicted to represent the same seed zone by the mid-century. For example, we suggest collecting seeds from the current blue climatype (Figure 4a), producing seedlings in the nursery and then reforesting sites in the blue climatype projected for the year 2060 (Figure 4b).
Ecological restoration considering climatic change: New assemblages of species as “novel” ecosystems
There are many ecosystem management practices that should consider climate change for planning and implementation. For some, such as forestry, that center on production and economic goals (Sarr, Puettmann, Pabst, Cornett, & Leonel, 2004), the selection of economically important species suitable for future climatic scenarios (as discussed above) will be sound. For others, such as ecological restoration and conservation, the role of assisted migration remains the subject of debate. Ecological restoration seeks to assist the recovery of ecosystems by creating suitable conditions for the reestablishment of structural and functional attributes, while conservation biology seeks to maintain the population viability and biotic integrity of ecosystems. Considering that the conditions at most locations will be different in the future, the goal of reestablishing the original ecosystem seems unrealistic. It will also be impossible to assist the migration of entire assemblages of native species that belong to any particular ecosystem in order to establish them elsewhere. It has therefore been argued that restoration techniques cannot prevent the negative effects expected from climate change, but merely help to ameliorate some of its effects (McCarty & Zedler, 2001) by providing techniques to establish key species and creating new assemblages of species that have been called “novel ecosystems” (Hobbs, Higgs, & Harris, 2009). In fact, different modeling approaches predict that novel ecosystems will form without human intervention; for example, boreal grassland-steppe in areas currently covered by Alaskan tundra (Chapin & Starfield 1997) or a novel ecosystem without a contemporary equivalent in the Yucatán peninsula, south-eastern Mexico (Rehfeldt et al., 2012).
Concerns about invasive species
It should be noted, however, that there are other opinions: that assisted migration would disrupt or displace local communities and that relocated populations would become invasive (Hewitt et al., 2011; Ricciardi & Simberloff, 2008; Seabrook, Mcalpine, & Bowen, 2011). The core of the disagreement is the level of management necessary in natural landscapes in order to accommodate climatic change. In the opinion of Seabrook et al. (2011), for example, natural landscape conservation should be the main goal and these landscapes should be left to respond to climate change naturally, even if extinctions do occur.
There is less disagreement over what Seabrook et al. (2011) call “production landscapes” (e.g. forests under intensive management for wood production or tree plantations) and urban landscapes, where the creation of novel ecosystems is a perfectly reasonable goal. A conceptual framework in which to clarify these management dilemmas is discussed by Pedlar et al. (2012) and grouped into a pragmatic operational forestry assisted migration approach compared to a “species rescue” conservationist approach.
Risks of inaction
There is concern that assisted migration would be designed based on possibly erroneous predictions of climatic change, given the inadequacies of modeling or uncertainty regarding the quantity of greenhouse gases that will be released in the future (McLachlan, Hellmann, & Schwartz, 2007). While valid, these concerns have led to inaction and it is therefore pertinent to ask the question: is inaction a better option? There are no reasons to believe that inaction would keep current tree populations intact. The massive and sudden decline of forest tree populations that has occurred with the mean annual temperature increase of around 1 °C as a result of the climate change already in place, seems to us a strong indication that the predicted temperature increases of 2, 3, 4 or 5 °C will cause a disastrous disruption to plant communities. There is a point where assisted migration would no longer be an option: if forest trees in the future become dead or too weak to produce healthy seeds, then it might be impossible or unaffordable to assist their migration in the future.
Strategies to face uncertainty
In order to reduce the risks of uncertainty regarding the best genotype for a given site, one option would be to plant a stock of seedlings with a wide array of genotypes (mixing seed sources that are local and presumably adapted to the future climate), encompassing wide genetic diversity, and simply let natural selection take its course. This would of course require planting higher densities than would normally be the case, in order to account for the mortality expected as a result of natural selection (Ledig & Kitzmiller, 1992).
Uncertainty related to the disparity of predictions for a given region among different global circulation models, and their combinations with different greenhouse effect gas emission scenarios (combinations named “model scenarios”), could be also approached from a different and complementary perspective. This uncertainty could be reduced if priority for assisted migration is given to locations where greatest agreement exists among model-scenarios, and less priority is given to regions with high disparity among predictions, and where the probability of failure is higher (Rehfeldt et al., 2012). The probability of failure persists, even with a perfect prediction of a match of genotypes and future environments. This is because one characteristic of climatic change is the enormous increase in variability among and within years of climatic patterns, particularly related to precipitation and heat waves (Hansen et al., 2012), while models are mainly generated with average values. It may therefore be necessary to repeat plantings in order to ensure some degree of success.
The probability of success increases if we aim to match a climatic scenario over a shorter time frame. To match in the present the climate predicted for 2090, instead of that for 2030 (e.g. moving 900 m upwards in altitude to compensate for an increase of, for example, 4.5 °C predicted for 2090, instead of moving 300 m upwards to compensate for the 1.5 °C increase predicted for 2030), would expose the seedlings to a much higher probability of frost damage (Sáenz-Romero & Tapia-Olivares 2008), without even considering that the temperature prediction for 2090 has more uncertainty than the prediction for 2030. This is one reason why we suggest matching with climates of 2030, or 2060 at most.
In case of strong opposition to assisted migration under the argument of uncertainty of future projections, at least we should be able to agree on the magnitude of past climate change (e.g., from the industrial revolution to the present). We could therefore initiate assisted migration efforts by migrating seed sources to mitigate for this recent past climate change.
Larix occidentalis massive assisted migration in British Columbia
One example of assisted migration in response to climate change is that of the western larch (Larix occidentalis Nutt.) in British Columbia, Canada, where the upward seed transfer guideline has been increased by 200 m within the natural range of the species and new seed zones have been delineated far north of the natural range of the species (O’Neill et al., 2008). This action has been based on recommendations by Rehfeldt and Jaquish (2010), among others. In many sites in central British Columbia, the western larch is being planted in mixed species plantations as a means by which to diversify natural stands and replace the lodgepole pine (Pinus contorta var. latifolia Douglas) stands that were wiped out by a massive outbreak of mountain pine beetle. These plantations have been very successful so far and there are plans to expand the program (Figure 5).

Figure 5 Assisted migration plantation of Larix occidentalis (western larch, yellow crowns at center of the photo, taken in October 2012) near Nakusp, British Columbia, Canada. The site originally consisted of Picea engelmannii Engelm. and Pinus contorta (narrow green crowns), but was severely damaged by an outbreak of mountain pine beetle. Dead trees were logged before establishment of the new plantation.
Urgent issues to address
Establishment of new common garden tests and small-scale assisted migration field tests is urgently required in order to accumulate sound scientific data and evidence to support assisted migration as a valuable management option over the long term. Examples of these are: a) the Assisted Migration Adaptation Trial (AMAT), where forty-eight seed sources from 15 tree species originating from British Columbia, Canada, and the north-western USA are being tested at 48 field test locations between the southern Yukon and northern California (Marris, 2009; O´Neill et al., 2012); b) a common garden experiment with 35 populations of coast Douglas-fir (Pseudotsuga menziesii var. menziesii [Mirb.] Franco), which was established at three disparate test sites with contrasting summer temperatures and precipitation throughout the states of Washington and Oregon, USA (Bansal, Harrington, Gould, & StClair, 2015); c) Quebec Province; featuring nine field tests of Picea glauca Voss, where seedlings originated from six first-generation and two second-generation seed orchards were established outside their local seed zones, moving them latitudinally and longitudinally, in a South-North and East-West oriented 3 x 3 grid arrangement, and d) four provenances, of each of three Mexican pines (Pinus pseudostrobus Lindl., Pinus leiophylla Schltdl. & Cham. and Pinus devoniana Lindl.) were planted in common garden field tests along an altitudinal gradient in Michoacán, Mexico (Castellanos-Acuña, Lindig-Cisneros, & Sáenz-Romero, 2015).
Re-examination of species and provenance tests conducted without an assisted migration philosophy but including the process nevertheless is a valuable source of data in some cases, but in others the chosen test sites do not include extreme climatic sites, making the fitting of reliable transfer or response functions difficult (Leites, Robinson, Rehfeldt, Marshall, & Crookston, 2012).
Regulatory norms to prevent or excessively regulate germplasm movement across political borders and protected area regulations that prohibit the introduction of non-local species need to be reexamined and relaxed where possible, especially when assisted migration would only imply the range expansion of a given species to higher altitudes or closer to the respective pole than the current natural distribution limit.
Conclusions
As a result of rapid climatic change, the shift in geographic location of suitable climatic habitat for forest tree species and populations is likely to cause considerable stresses on natural tree populations. Due to the physical limits to natural migration, tree populations will be unable to keep up with the moving target that their suitable climatic habitat will become. Such decoupling between natural populations and the climate for which they are adapted is likely to cause massive forest decline. As a management option, we suggest assisted migration, which is the realignment of natural populations to the climate for which they are adapted, through reforestation in sites where their suitable climate is predicted to occur in the future. This represents an active management option that aims to provide healthy tree ecosystems in the future.