List of acronyms (in order of appearance in the text)
Abbreviation | Meaning | |
AA | Alkali activator | |
AAC | Alkali-activated cement | |
MK | Metakaolin | |
FA | Pulverized fly ash | |
BFS | Blast furnace slag | |
CW | Ceramic waste | |
NS | Nanosilica | |
DE | Diatomaceus earth | |
FCC | Spent fluid catalytic cracking catalyst | |
WG | Sodium silicate | |
WD | Wasted diatomite | |
SF | Silica fume | |
GW | Glass waste | |
RHA | Rice husk ash | |
SCSA | Sugar cane straw ash | |
SCBA | Sugar cane bagasse ash | |
BLA | Bamboo leaf ash | |
BL | Bayer Liquor | |
RM | Red mud | |
ACS | Alkaline cleaning solution | |
OBA | Olive-stone biomass ash | |
ABA | Almond shell biomass ash | |
HCWA | High calcium wood ash | |
CCA | Corn cob ash |
1. Introduction
The term "circular economy" has been established in most proposals on the development of humanity in global challenges (climate change, biodiversity, waste, pollution). This circular economy focuses on a production+use model that prioritizes the reduction, reuse, repair, recycling, recovery and valorization of products. Thus, products’ life cycles should be extended for as long as possible by minimizing the use of natural resources and energy, and reducing waste generation and environmental pollution.
Circularity must be extended to each development, scientific, technological and human area. As many manufacturing processes are related to chemical reactions and processes are associated with pollution and waste management phenomena, the term "circular chemistry" becomes especially important (Keijer et al., 2019; Mohan and Katakojwala, 2021). Chemistry is the fundamental science for circular economy, where strategies focus on chemical innovation at atomic, molecular and structural levels.
In the construction field, and specifically in concrete technology where concrete is the most widely used material by humanity after water, technological breakthroughs must be developed according to circular chemistry. As a key binder material in concrete preparation, cement is a synthetic material made from various components. It is made up of a set of chemical compounds which, by reacting with water, develop other chemical products responsible for binders' technological properties.
In ancient times, nonhydraulic binders based on gypsum and hydrated lime were prepared by transforming natural materials. These binders were reacted with water and/or carbon dioxide (CO2) to produce stable resistant materials in certain environments. Roman engineers introduced pozzolanic materials, mainly ashes of volcanic origin, into mixtures with hydrated lime, which involved a transcendental chemical turnabout (Pavía and Caro, 2008). Many centuries later, these ancient materials were taken up again to develop new cements with pozzolanic additions.
In the late eighteenth and early nineteenth centuries, chemical processes were implemented to manufacture hydraulic binders, which culminated with Joseph Aspdin's patent in 1824: it started the Portland cement period by drastically reducing the use of hydrated lime and gypsum. Cement is the material that has been employed to make many buildings and infrastructures from that time to the present-day (Hall, 1976). The development of high-temperature chemical processes (clinkerization) allowed high-performance cements with good durability. Knowledge of the chemical reactions of Portland cement hydration and their interaction with concrete components and the environment (humidity, CO2, chlorides, etc.) allowed the evolution of the characteristics of new commercial cements based on Portland cement clinker.
In recent decades, concerns about greenhouse gases emissions from manufacturing Portland cement, perseverance to reduce the use of nonrenewable raw materials, and the requirement to improve concrete’s technical performance have led the chemistry of materials toward new more sustainable, complementary and circular paths (Phair, 2006). Intensive research has been carried out on cements with a higher proportion of pozzolanic and hydraulic additions, belite cements, calcium sulfoaluminate cements, magnesia cements and alkali-activated cements (AACs).
This paper focuses on AACs, specifically on alkaline activators (AAs), their impact in carbon footprint terms, and the alternatives involved in applying circular chemistry. This paper is divided into an initial section about the basic concepts of the chemistry of AACs, followed by a comparative evaluation of the carbon footprint associated with different conventional AACs, starting with Portland clinker-based cements and finishing with a description of alternative materials of the residual origin that can be used to produce alternative AAs, along with carbon footprint-related aspects.
2. Nature of alkali-activated cements and the origin of components
Alkali-activated cements (AACs) are formed by combining two components ("two-parts" technology): the major component is the precursor, which contains SiO2-Al2O3 (e.g., metakaolin - MK, fly ash from coal-fired power plants - FA). Sometimes it can also contain significant amounts of CaO (blast furnace slag - BFS). The other minor component is a highly alkaline aqueous solution (sodium or potassium hydroxides, silicates and carbonates). The mixture of these two components produces cementitious substances like the alkaline aluminosilicate hydrated type (N(K)-A-S-H) or, if calcium is present, the calcium aluminosilicate hydrated type (C-A-S-H). Gels of a hybrid nature between the two previous ones, C(N,K)-A-S-H, can also be produced.
From the circular chemistry and sustainability points of view, the marked advantage of AACs lies in the fact that it is often possible to use waste from other anthropogenic industrial and agricultural activities, as well as ceramic waste (CW), BFS and FA. Circularity is not possible in other cases because the precursor is specifically manufactured, such as MK (kaolin calcination at 800ºC). In AACs, the application of very high temperatures is avoided, which occurs in the clinkering process of Portland cement (1450ºC). The main disadvantage of AACs is that the activator is a chemically synthesized substance: e.g., hydroxides NaOH and KOH, carbonates Na2CO3 and K2CO3, silicates Na2SiO3 (waterglass) and K2SiO3. The manufacturing of these substances involves employing natural resources and energy, and following chemical processes that have a significant impact and move away from circularity. In recent years, the major development of AACs has taken place, when two components have been combined into one ("one-part" technology) so their application can follow the same model of traditional cements: just add water to "one-part" cement.
3. Carbon footprint in alkaline activation cements
It is well-known that CO2 emissions are related to Portland cement clinker manufacturing, i.e. the carbon footprint is very large. The process involves the emissions of 850-1200 kg of CO2/ton-clinker, which depends mainly on the applied technologies and the effectiveness of the heat recovery and insulation of industrial elements. CO2 emissions can be assigned to two main components: the chemical component on the one hand, and the energy component on the other.
The chemical component is due to limestone decarbonation. For a clinker containing 64% CaO, the amount of CO2 from the chemical component (CO2q) is approximately 500 kg of CO2/ton-clinker. The amount of CO2 from the energy component (CO2fd) associated with fuel and electrical energy use approximately equals CO2q (Luukkonen et al., 2016). Relatively speaking, we can establish a value of 100 for the CO2 emissions associated with cement with 95% clinker (cement type CEM I in the European nomenclature), with a contribution of 50 for CO2q and 50 for CO2fd (See Figure 1, CEM I). For cements with mineral additions, each contribution proportionally reduces: Figure 1 shows the CEM II/A (with 20% addition) and CEM II/B (with 35% addition) cases. Currently in Europe, work is being done on the standardization of CEM II/C-M cement (EN 197-5, 2021), which includes a mixture of additions (M) up to 50% (see Figure 1). For cements with additions, we must consider additional components like energy used to prepare additions (sieving, grinding, drying) in either independent or mixing processes with non-ground clinker. These additional components are named “conditioning”.
Synthetic AAs have certain associated CO2-equivalent emission values (CO2-e, kgCO2/kg-reagent), and these values very much depend on the industrial process and the technology used in synthesis (see Table 1).

Figure 1 Comparison of the relative emissions of Portland cement clinker-based cements: CEM I, CEM II/A (with 20% addition), CEM II/B (with 35% addition) and CEM II/C-M (with 50% addition). Relative scale takes 100 for CEM I.
Table 1 CO2 emissions for some alkaline reagents (taken from: www.winnipeg.ca/finance/findata/matmgt/documents/2012/682-2012/682-2012_Appendix_H-)
Reagent | CO2-e kgCO2/kg-reagent |
---|---|
NaOH | 1.12 |
KOH | 1.94 |
Na2CO3 | 0.59 |
K2CO3 | 2.38 |
Na2SiO3 | 1.64 |
Metakaolin (MK)-based AACs require significant amounts of activator, especially sodium silicate SS (Weil et al., 2009). Moreover, the manufacture of MK requires the calcination of kaolin at 800ºC, which involves significant energy use. An estimated calculation of the associated emissions for an MK-based AAC is shown in Figure 2: compared to CEM I, no significant advantages appear. Obviously, the lower the sodium silicate use in the dose, the more advantageous it is. Fly ash (FA)-based AACs also require vast amounts of activator. To achieve good geopolymerization development, high-temperature curing is required. Hence additional energy use is to be considered in their application (curing process). Figure 2 depicts how the corresponding relative CO2 emissions are also very high. In the two previous cases based on MK and FA, the calcium content of these precursors is low, which requires a large amount of activators. With calcium-rich precursors, such as BFS, a smaller amount of activators is needed. In addition, the precursor can be activated with NaOH solution without having to resort to alkali silicate (SS or potassium silicate). Figure 2 shows the marked advantage of AAC-BFS over the relative carbon footprint compared to the previously discussed AACs. Slag requires a milling process (precursor conditioning) to improve its reactivity in the alkaline medium.
4. Alternative alkaline activators
As seen in the previous section, the contribution of AAs to the carbon footprint of AACs is most relevant. This means that improvements in this aspect should focus on minimizing the use of this component. One choice would be to center on improving the chemical processes of the synthesis of chemical reagents so that the associated CO2-e lowers. The other option, which is more suitable for circular economy and chemistry, is the valorization of agricultural and industrial waste for this purpose. In recent years, there have been more scientific waste valorization reports about achieving efficient activators with a smaller associated carbon footprint. These publications contain a variety of options, which can be classified as the following groups:
Alternative activators based on silica: related to alkaline silicates, where an alkaline hydroxide is reacted with a rich-silica raw material, which is totally or partially dissolved
Alternative activators based on alkali: in this case, the employed residual material is an alkaline, sodium or potassium-based substance
Combined silica-AA systems
4.1. Alternative activators based on silica.
There are different strategies for this group: on the one hand, the use of natural silica-based resources that can be dissolved under distinct conditions; on the other hand, the availability of ashes from biomass combustion with high SiO2 percentages; finally, the possibility of using some industrial waste rich in potentially reactive silica.
4.1.1. Silica-based activators from natural resources.
In this section, references related to diatomaceous earth and olivine can be found.
Diatomaceous earth (DE) is a silica-rich material (generally SiO2>80%) formed by the skeletons of diatoms, unicellular algae, which have been deposited over millions of years. This sedimentary rock presents silica in the amorphous state, which can be dissolved under alkaline conditions. Font et al. (2018) presented a study in which DE was dissolved in NaOH solution in a thermally insulated vessel. The dissolution of NaOH pellets in water increases the temperature of the aqueous medium that, in turn, enhances amorphous silica dissolution. The obtained results show the prepared activator’s good performance. So these authors used this activator for the FCC precursor (fluid catalytic cracking catalyst residue). In mortars, mechanical strengths of 30 MPa after 7 curing days at room temperature were obtained without having to employ high-temperature curing.
Olivine, (Fe,Mg)2SiO4, is a natural material that can be used to obtain nanosilica from a dissolution process with concentrated sulfuric acid and subsequent filtration (Gao et al., 2017). These authors utilized a solution prepared with nanosilica (NS) and NaOH to show that reactivity was similar to commercial sodium silicate (waterglass, SS). They applied this activator to a mixture of BFS and FA at the 70/30 ratio. The mechanical strength of concretes yielded about 57-68 MPa at 7 days and 72-82 MPa at 28 days, depending on the silica modulus (SiO2/Na2O molar ratio) used in the activating solution.
4.1.2. Silica-based activators from industrial waste.
This section offers examples based on residual DE, silica fume (SF) and glass waste (GW).
DE is frequently used as a component of liquid filter media, especially beer and wine. At the end of the filtration process, the residual DE (WD) contains significant amounts of potentially soluble amorphous silica. WD from beer filtrate (WD-B) was used to prepare alkaline activators with NaOH (Mejía et al., 2016). This activator was combined with a precursor consisting of a mixture of FA and MK (70/30). Paste was cured at 60ºC for 24 h and subsequently cured at room temperature for up to 360 days. The compressive strength values within the range 7-360 days came close to 35 MPa. WD from wine filtrate (WD-W) was studied by Font et al. (2018). These authors evaluated the performance of the activator based on this residue. They observed a substantial improvement when WD-W was calcined at 650ºC to remove the organic matter from the filtration process. The mechanical compressive strength at 28 days of the activated FCC mortars was about 20 MPa for the system with no prior WD-W calcination, and was 40 MPa for the system with calcination.
SF is a well-known and excellent pozzolan in Portland cement systems thanks to its marked fineness and high amorphous silica content. This residue, from the silicon and ferrosilicon alloys industry, can be used as raw material to produce activators. Rouseková et al. (1997) studied BFS activation with a mixture of SF and NaOH, and obtained good results. Bernal et al. (2012) studied the SF/NaOH activator in MK+BFS systems to show that silica from SF can be incorporated into the geopolymerization reaction for very short times. Mixtures of C-A-S-H, N-A-S-H, or even of N-(C)-A-S-H cementitious gels, were formed.
For GW, various chemical GW compositions can be found, although the most notable one is the amorphous state and a high percentage of silica. Since 2014, the potential of GW for manufacturing activators has been studied. The SiO2 percentages in GW fall within the 65-80% range with significant CaO and Na2O contents (Liu et al., 2019). Several approaches have been performed to prepare the AA, as shown in Figure 3. They include melting, hydrotherma, and thermochemical methods. It has been shown that the nature and rate of formation of cementitious hydrates depend primarily on the precursor type.
4.1.3. Silica-based activators from biomass ash.
This section contains the largest number of alternative activators. Biomass combustion sometimes generates the formation of a significant amount of ash (1-4%, in mass). The most significant examples are waste from rice husks, sugar cane production and bamboo leaves.
Rice husk ash (RHA) is the most widely studied. Many works have been published about using RHA to produce sodium silicate. The first bibliographic reference dates back to 2010 (Bejarano et al., 2010). The authors proposed a hydrothermal process during which they analyzed different variables: NaOH:RHA ratio, treatment time, reaction temperature and water:RHA ratio. The conclusions on the optimum parameters were NaOH:RHA=2, water:RHA=10, 100ºC treatment for 120 minutes. With these proportions, the research group used this activator type in the alkaline activation of several common precursors (Mejía et al., 2016). Bouzón et al. (2014) applied an activator obtained by refluxing a mixture of NaOH solution with partially amorphous RHA, and by using FCC as a precursor to prepare mortar. Systems with excellent mechanical performance were obtained, but the authors observed that the activator was more reactive if ground RHA was used during the hydrothermal process (around 60 minutes, compared to 120 minutes for the non-ground sample). Other authors have devised methods and applications for this material (including Tong et al., 2018; Rajan and Kathirvel, 2021). Villca et al. (2021) applied an alternative RHA-based activator, produced with no energy use, but by simply heating the dissolution of NaOH pellets to dissolve the amorphous silica of RHA. This activator was applied for the first time to binary systems of lime/pozzolan and geopolymer.
Sugarcane production generates a waste product, sugarcane straw, which is usually left in fields. Using this biomass can be beneficial in energy recovery terms. The generated waste, sugarcane straw ash (SCSA), contains large amounts of silica (Moraes et al., 2018). This research demonstrated that the procedure which takes advantage of the dissolution heat of NaOH to dissolve silica is perfectly viable for SCSA and treatment should not exceed 24 h due to the gelation of the activator. Sugarcane bagasse ash (SCBA) has also been employed (Tchakouté et al., 2017) in the geopolymerization of MK. The prepared binder reached 33 MPa at 28 curing days.
Finally, bamboo leaf ash (BLA), with a SiO2 percentage close to 80% (Roselló et al., 2015), is also an interesting option. BLA has been used by a thermochemical process at 300-330ºC (Vinai et al., 2021). The activator was utilized for the FA/BFS system (60/40). Compared to the SS+NaOH activator, reactivity was lower on the first 7 curing days, and the same strengths were obtained (around 43 MPa) at 28 days.
4.2. Alternative activators based on alkali.
Human activity also produces alkaline waste of both industrial and agricultural origins.
4.2.1. Alkali-based activators from industrial activities.
We can find some waste related to metallurgical activity, such as Bayer liquor, alkaline cleaning solutions and red mud.
Bayer liquor (BL) is a caustic solution prepared with NaOH, used to extract aluminum from bauxite. After filtration, the resulting solution is reusable, but has to be eventually discarded when its efficiency decreases. The waste is rich in sodium aluminate and has been employed for FA activation under different conditions (Jamieson et al., 2016).
Red mud (RM) is the solid waste generated while extracting aluminum from bauxite. It is very rich in iron oxides and silica. This sludge has a pH between 9.7 and 12.8, and has been used to activate FA (Choo et al., 2016), although the achieved strength does not exceed 2 MPa.
The last example in this category is alkaline cleaning solution (ACS), which is usually produced as waste when cleaning aluminum surfaces. It is rich in sodium hydroxide and sodium aluminate. Fernández-Jiménez et al. (2017) employed ACS at a NaOH concentration of approximately 5M to activate ground FA and GW powder. In both cases, mechanical strengths were around 10-16 MPa for FA and 2-10 MPa for GW (cured for 20 h at 85°C).
4.2.2. Alkali-based activators from agricultural waste.
Some agricultural waste is considered to be biomasses for energy production, and the resulting ashes are alkaline in nature. This alkaline nature is determined by the presence of calcium, especially that of potassium. This applies to olive stone ash (OBA), almond shell ash (ABA), corn cob ash (CCA), and high calcium wood ash (HCWA). The great advantage of using these ashes is that they allow “one-part" cements to be prepared as they are solid materials.
The first study on employing OBA in AAC was presented by Font et al. (2017). BFS was activated with OBA and compared to activation by means of chemical reagent KOH. The result showed that the compressive mechanical strength for the BFS/OBA system was higher (29.9 MPa vs. 16.9 MPa). Subsequent studies (De Moraes Pinheiro et al., 2018) showed the formation of cementitious gels of the C(K)-S-H, C(K)-S-A-H type, in addition to small amounts of hydrotalcite. Subsequently, Alonso et al. (2019) studied ashes from an olive biomass: both FA and bottom ashes. They observed that the alkalinity of these ashes was not sufficient to activate FA, but sufficed to activate BFS. Payá et al. (2019) employed the BFS/OBA system to produce dolomitic-based compacted earth blocks. The compressive strength of about 12 MPa for compacted blocks was obtained after 9 curing days under plastic film (to prevent water evaporation and to maintain optimal hydration conditions). The resulting blocks showed excellent resistance underwater.
Soriano et al. (2020) were the first to study the potential of almond shell ash (ABA). They found that the percentage of K2O for ABA was higher than for OBA (46.98% vs. 32.16%). They detected the presence of several calcium and potassium carbonate minerals (K2Ca(CO3)2), such as fairchildite and bütschilite, in addition to calcite (CaCO3) portlandite (Ca(OH)2) and arcanite (K2SO4). This ash performed well when combined with BFS.
Several authors have investigated the use of HCWA. Of these authors, Ban et al. (2014) characterized ash and applied it as a solid activator for FA. Ash presented a high proportion of CaO (61%) and a smaller amount of K2O (12%). The found calcium salts were calcite, portlandite and hydroxyapatite.
Rachis or crown CCA has also been used for MK activation (Peys et al., 2016), and 30 MPa compressive strength has been achieved for samples with a CCA:MK ratio of 1:2.
4.3. Combined silica-alkali systems.
AAC based on the use of alkaline ash can reduce impacts because, in some cases, both the precursor and activator come from waste. However, mechanical performance is often not very good due to the activator’s silica deficiency. Thus, the development of activators containing silica and alkali could minimize this effect. Font et al. (2020) designed AAC with the BFS-OBA-RHA ternary system, in which the activator was prepared by a hydrothermal reaction between RHA and OBA at 65ºC for 24 h. For a BFS-OBA-RHA dose of 9/4.5/1, 35.0, 46.2 and 67.4 MPa were obtained for mortars respectively cured for 7, 28 and 90 days at 20ºC.
4.4. Classification of biomass ashes according to their reactivity.
Employing biomass ashes is an interesting alternative for synthesizing sustainable activators. The ternary diagram in Figure 4 illustrates an extended version of the Vassilev diagram (Vassilev et al., 2010) of the biomass ashes classification. Reactivity zones have been modified, and different ashes have been represented. In zone S (top of the diagram) we see a set of ashes that are potentially very acidic and can supply a high percentage of silicates to alternative activators: RHA, BLA and sugar cane bagasse ash (SCBA). In an intermediate situation (Zone K-MA), we find sugar cane straw ash (SCSA) whose composition contains other elements that reduce its contribution to silicate formation. Finally in low-acid materials (Zone K-LA), we find CCA, with limited silicon contribution and a high proportion of potassium. At the bottom of the diagram, where more alkaline oxides are involved, basically CaO (far left) and K2O (far right), three zones stand out: the "C" zone, which is characteristic of ashes with high calcium content, such as HCWA; the "K" zone, with a high proportion of potassium oxide, as in ABA; finally, the intermediate "CK" zone, where OBA is found.
5. Carbon footprint of alkali-activated cements using alternative alkaline activators
BFS activation with synthetic activators results in relatively low CO2 emissions (Figure 2). However, this can improve if alternative activators are employed. By way of example, the system with OBA is analyzed. In addition to waste recovery (and also energy recovery for biomass), the associated CO2 can also slightly drop. With the BFS-OBA system (Figure 5), the conditioning of the precursor is logically the same, and only the conditioning of the activator (grinding in this case) has to be taken into account. The associated CO2 emissions for the BFS-OBA-RHA system are higher because the activator must be conditioned by the hydrothermal treatment of the OBA-RHA mixture. However, this new activator enables higher mechanical strength to be achieved and, thus, extra CO2 emissions can be compensated by the obtained material’s mechanical performance.
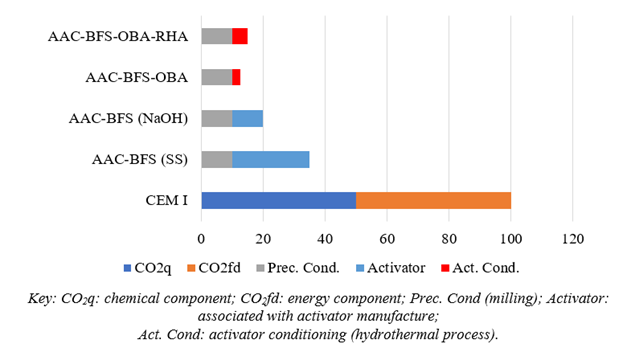
Figure 5 Comparison of relative emissions of the CEM I cements and AAC produced with alternative activators and the blast furnace slag (BFS) Precursor. Commercial activators: sodium hydroxide (NaOH) and sodium silicate (SS); alternative activators, olive stone ash (OBA) and rice husk ash (RHA). Relative scale takes 100 for CEM I.
6. Conclusions
The development of AACs requires the availability of both, suitable precursors and activators. The capacity to obtain precursors in sufficient quantities and locations to completely replace Portland cement is probably insufficient. Therefore, it is not easy to consider AACs to be the only solution. Their production must be taken as an alternative to Portland cements and other cement types (belite, calcium sulfoaluminate, magnesia-based). The main fact is that there are places where potentially marketable precursors are generated (ceramics, construction waste, different metallurgical slag types), and all possibilities should be exploited. The same applies to alternative activators: synthetic chemical reagents can be replaced where agricultural or industrial activities are performed, which can generate suitable components to prepare alternative activators. This circumstance may be particularly interesting in developing societies where it is not easy (basically economic) to use Portland cement, but where there are alternative resources to manufacture AACs (construction waste, biomass, etc.).
The following conclusions about alternative activators stand out:
a) AACs do not always result in the drastic reduction of associated CO2 emissions. In some cases, the required quantities of chemical reagents represent a comparable carbon footprint to that of Portland cements
b) Precursors like BFS require smaller quantities of activator, which results in lower CO2 emissions than for other precursors like FA or MK
c) Alternative activators can be of agricultural or industrial origin but, in any case, require complete characterization, including both necessary preconditioning studies and the potential problems of their use (presence of harmful chemical components for some applications, such as chlorides)
d) Silica-based waste cannot be independently used because of its high acidity (mainly silica). Alkali-based waste can be independently employed. Combining both waste types can improve the mechanical behavior of AACs and enhance their uses
e) The use of solid waste as alternative activators allows "one-part" cements to be produced. Additionally, the precursor and activator can be ground, which improves the intimate mixture of both components.